#Mitochondrial permeability transition pore(mPTP)
Explore tagged Tumblr posts
Text
Mitochondrial Dysfunction in the Pathogenesis of Parkinson’s Disease
Introduction
Parkinson's disease (PD) is a progressive neurodegenerative disorder primarily affecting motor function due to the selective degeneration of dopaminergic neurons in the substantia nigra pars compacta. The pathogenesis of PD is multifactorial, with emerging evidence pointing to mitochondrial dysfunction as a pivotal event in the onset and progression of the disease. This article provides a comprehensive technical analysis of the role of mitochondrial dysfunction in PD, focusing on key molecular mechanisms, genetic factors, and potential therapeutic strategies.
Mitochondria and Their Cellular Roles
Mitochondria are essential organelles that generate the majority of the cell's ATP via oxidative phosphorylation in the electron transport chain (ETC). In addition to their role in energy production, mitochondria are involved in maintaining cellular homeostasis by regulating calcium signaling, apoptosis, and reactive oxygen species (ROS) production. The proper functioning of mitochondria is crucial for neurons, particularly dopaminergic neurons, which have a high metabolic demand.
Mitochondrial Dysfunction and Parkinson's Disease Pathogenesis
Mitochondrial dysfunction in PD primarily manifests through alterations in mitochondrial bioenergetics, increased oxidative stress, defective mitophagy, and calcium dysregulation. These abnormalities converge on exacerbating neuronal injury, particularly in dopaminergic neurons.
1. Impaired Mitochondrial Complex I Activity
One of the hallmark features of mitochondrial dysfunction in PD is the impairment of mitochondrial complex I, the first enzyme complex in the ETC. Complex I is responsible for transferring electrons from NADH to ubiquinone, a critical step in ATP synthesis. Studies consistently show that PD patients exhibit significant reductions in complex I activity in the substantia nigra, which leads to defective ATP production. This mitochondrial dysfunction results in energy deficits, rendering dopaminergic neurons more susceptible to stressors.
Inhibition of complex I activity is not limited to genetic mutations; environmental toxins such as rotenone and paraquat, which inhibit complex I, have been implicated in Parkinsonian syndromes. Furthermore, complex I dysfunction increases the production of ROS, exacerbating oxidative stress in neurons and contributing to mitochondrial damage.
2. Oxidative Stress and ROS Generation
Mitochondria are both the primary source and target of ROS. The process of oxidative phosphorylation inevitably generates ROS as byproducts, particularly superoxide anion, hydrogen peroxide, and hydroxyl radicals. Under normal conditions, ROS are detoxified by endogenous antioxidant systems. However, in PD, mitochondrial dysfunction leads to an imbalance between ROS production and the cell’s antioxidant defenses.
The substantia nigra, which is particularly vulnerable in PD, is exposed to elevated ROS levels due to the high metabolic rate of dopaminergic neurons and the catabolism of dopamine, which generates additional ROS via the action of monoamine oxidase (MAO). Accumulation of ROS results in lipid peroxidation, protein misfolding, and mitochondrial DNA (mtDNA) mutations, all of which contribute to neuronal death and the progression of Parkinson’s pathology.
3. Mitophagy and Dysfunctional Quality Control Mechanisms
Mitophagy, a selective autophagic process that removes damaged or dysfunctional mitochondria, is crucial for maintaining mitochondrial quality and function. In PD, mitophagy is impaired, leading to the accumulation of damaged mitochondria within neurons. The PINK1-parkin pathway plays a pivotal role in the initiation of mitophagy. PINK1, a mitochondrial kinase, accumulates on depolarized mitochondria and recruits the E3 ubiquitin ligase parkin, which ubiquitinates outer mitochondrial membrane proteins to tag them for autophagic degradation.
Mutations in the PINK1 and parkin genes, which are associated with autosomal recessive forms of PD, disrupt this process and contribute to the accumulation of dysfunctional mitochondria. This failure to remove damaged mitochondria exacerbates oxidative stress and promotes the activation of apoptotic signaling pathways. As mitochondrial dysfunction progresses, neuronal survival becomes increasingly compromised, accelerating disease progression.
4. Calcium Homeostasis and Mitochondrial Regulation
Mitochondria play a critical role in buffering cytosolic calcium levels. Neurons, due to their high metabolic activity, are particularly dependent on mitochondrial calcium buffering to prevent cytotoxic calcium overload. However, in PD, mitochondrial dysfunction leads to impaired calcium handling, resulting in an increase in cytosolic calcium concentrations.
Elevated calcium levels activate a variety of calcium-dependent enzymes, such as calpains and phospholipases, that further damage cellular structures. Additionally, excessive calcium in mitochondria can activate the mitochondrial permeability transition pore (mPTP), leading to mitochondrial depolarization, the release of pro-apoptotic factors such as cytochrome c, and eventual cell death.
Genetic Factors in Mitochondrial Dysfunction in PD
Genetic mutations that directly affect mitochondrial function have been identified in familial forms of PD. These mutations often impair mitochondrial dynamics, quality control, and bioenergetics, contributing to the pathogenesis of the disease.
PINK1 and Parkin Mutations: Mutations in the PINK1 gene and the parkin gene, both involved in the regulation of mitophagy, lead to impaired mitochondrial quality control. PINK1, a serine/threonine kinase, normally accumulates on damaged mitochondria and recruits parkin to initiate mitophagy. Loss of PINK1 or parkin function results in the accumulation of dysfunctional mitochondria, contributing to neuronal degeneration.
LRRK2 Mutations: The LRRK2 gene encodes a large protein kinase involved in multiple cellular processes, including mitochondrial dynamics and autophagy. Mutations in LRRK2 are the most common genetic cause of PD, particularly in late-onset forms. LRRK2 is implicated in the regulation of mitochondrial fission and fusion, processes that control mitochondrial morphology and function. Dysregulation of these processes leads to the fragmentation of mitochondria, impaired mitochondrial function, and increased susceptibility to oxidative stress.
Alpha-Synuclein and Mitochondrial Interaction: Alpha-synuclein, the protein most notably associated with Lewy body formation in PD, has also been shown to interact with mitochondrial membranes. Aggregation of alpha-synuclein disrupts mitochondrial dynamics, leading to decreased mitochondrial respiration and increased ROS production. This interaction exacerbates mitochondrial dysfunction and accelerates neurodegeneration.
Environmental Toxins and Mitochondrial Dysfunction
Environmental exposures, particularly to pesticides like rotenone and paraquat, have been shown to inhibit mitochondrial complex I, leading to oxidative stress and mitochondrial dysfunction. These toxins induce PD-like symptoms in animal models, supporting the hypothesis that environmental factors contribute to the pathogenesis of the disease.
Therapeutic Approaches Targeting Mitochondrial Dysfunction
Given the central role of mitochondrial dysfunction in PD, therapeutic strategies aimed at restoring mitochondrial function are being actively explored. These include:
Antioxidant Therapies: Antioxidants such as coenzyme Q10 (CoQ10) have been proposed to alleviate oxidative stress by scavenging ROS. CoQ10 functions as an electron carrier in the ETC and may help restore mitochondrial bioenergetics in PD. Clinical trials, however, have shown mixed results, necessitating further research.
Gene Therapy: Gene therapy approaches aimed at correcting genetic defects that impair mitochondrial function are under investigation. For example, restoring PINK1 or parkin function in neurons may enhance mitophagy and mitigate mitochondrial damage.
Mitochondrial Replacement Therapy: Mitochondrial replacement or mitochondrial transplantation holds promise as a therapeutic strategy for restoring mitochondrial function in PD. Early-stage studies are exploring the feasibility of mitochondrial transplantation into dopaminergic neurons to restore cellular function.
Exercise and Lifestyle Interventions: Regular physical exercise has been shown to stimulate mitochondrial biogenesis and improve mitochondrial function. Exercise-induced upregulation of mitochondrial regulators such as PGC-1α may provide neuroprotective benefits in PD by enhancing mitochondrial turnover and reducing oxidative damage.
Conclusion
Mitochondrial dysfunction is a central event in the pathogenesis of Parkinson's disease, contributing to the degeneration of dopaminergic neurons through mechanisms such as impaired mitochondrial complex I activity, oxidative stress, defective mitophagy, and disrupted calcium homeostasis. Genetic mutations in key mitochondrial regulators such as PINK1, parkin, and LRRK2 exacerbate these defects, while environmental toxins further contribute to mitochondrial damage. Targeting mitochondrial dysfunction through antioxidant therapies, gene therapy, and lifestyle interventions holds promise for mitigating the progression of Parkinson's disease. Understanding the intricate molecular mechanisms linking mitochondrial dysfunction to neurodegeneration in PD will be crucial for developing effective therapeutic strategies.

#Mitochondrial dysfunction#Parkinson’s disease (PD)#Dopaminergic neurons#Substantia nigra#Complex I activity#Oxidative stress#Reactive oxygen species (ROS)#Mitophagy#PINK1#Parkin#Mitochondrial DNA (mtDNA)#Calcium homeostasis#Mitochondrial permeability transition pore(mPTP)#LRRK2#Alpha-synuclein#Mitochondrial dynamics#Genetic mutations in PD#Environmental toxins#Rotenone#Paraquat#Coenzyme Q10 (CoQ10)#Antioxidant therapy#Gene therapy#Mitochondrial replacement therapy#Exercise and mitochondrial biogenesis#Neurodegeneration#Parkinsonian syndromes#Mitochondrial quality control#Mitochondrial fission and fusion#Neuroprotective therapies
0 notes
Link
Melatonin as Mitochondrial Medicine
The findings of this new study lend additional weight to the evidence that melatonin prevents age-associated disease through its impact on mitochondrial health.19
This should not be surprising, considering the highest concentrations of melatonin inside of cells is found in the mitochondria, which suggests an important natural role for its effects on energy production and cellular integrity.32
Indeed, melatonin is known for its ability to extend the lifespan of multiple species, from insects to mammals. This effect is accomplished through melatonin’s ability to protect mitochondria, promote longevity-associated proteins such as SIRT1, and reduce oxidative stress that can induce mitochondrial destruction.14-21
Specifically, melatonin can:
Prevent age-related mitochondrial dysfunction in brain cells, with the potential to slow or prevent neurodegenerative diseases,4,8-10
Prevent death of skeletal muscle cells through supporting mitochondrial energy production,5
Protect heart muscle cells following loss of blood flow (ischemia) during and after a heart attack,6
Improve mitochondrial function, and hence, energy utilization, in fat tissues of animal models of diabetes and obesity,11
Alleviate fatty liver disease by protecting liver mitochondria in similar animal models,12
Improve function of smooth muscle cells in intestines, which often slows down during aging as their energy supplies are threatened.2
With its newly-discovered ability to support the CNPase enzyme, and the resulting prevention of MPTP formation, melatonin helps preserve youthful function in every tissue in the body.
And since most human cells and tissues contain mitochondria, that translates to a vital protective effect of melatonin in all body organs and systems.
Summary
Loss of mitochondrial function is a fundamental contributor to aging in every cell, tissue, organ, and body system in humans.
A landmark study published in early 2017 has shown that melatonin supplementation supports youthful mitochondrial function by preventing the expression of an opening, or pore, or “hole” in mitochondrial membranes that would otherwise degrade their ability to generate energy.
This results in enhanced mitochondrial function, and a reduction in age-related diseases—and it goes a long way to explaining melatonin’s known longevity-promoting properties.
By supplementing with melatonin, we can preserve youthful energy supplies by providing protection for the body’s main energy source.
If you have any questions on the scientific content of this article, please call a Life Extension® Wellness Specialist at 1-866-864-3027.
References
Ganie SA, Dar TA, Bhat AH, et al. Melatonin: A Potential Anti-Oxidant Therapeutic Agent for Mitochondrial Dysfunctions and Related Disorders. Rejuvenation Res. 2016;19(1):21-40.
Martin-Cano FE, Camello-Almaraz C, Acuna-Castroviejo D, et al. Age-related changes in mitochondrial function of mouse colonic smooth muscle: beneficial effects of melatonin. J Pineal Res. 2014;56(2):163-74.
Paradies G, Paradies V, Ruggiero FM, et al. Protective role of melatonin in mitochondrial dysfunction and related disorders. Arch Toxicol. 2015;89(6):923-39.
Petrosillo G, Fattoretti P, Matera M, et al. Melatonin prevents age-related mitochondrial dysfunction in rat brain via cardiolipin protection. Rejuvenation Res. 2008;11(5):935-43.
Hibaoui Y, Roulet E, Ruegg UT. Melatonin prevents oxidative stress-mediated mitochondrial permeability transition and death in skeletal muscle cells. J Pineal Res. 2009;47(3):238-52.
Petrosillo G, Colantuono G, Moro N, t al. Melatonin protects against heart ischemia-reperfusion injury by inhibiting mitochondrial permeability transition pore opening. Am J Physiol Heart Circ Physiol.2009;297(4):H1487-93.
Petrosillo G, Moro N, Ruggiero FM, et al. Melatonin inhibits cardiolipin peroxidation in mitochondria and prevents the mitochondrial permeability transition and cytochrome c release. Free Radic Biol Med.2009;47(7):969-74.
Jou MJ. Melatonin preserves the transient mitochondrial permeability transition for protection during mitochondrial Ca(2+) stress in astrocyte. J Pineal Res. 2011;50(4):427-35.
Ozturk G, Akbulut KG, Guney S, et al. Age-related changes in the rat brain mitochondrial antioxidative enzyme ratios: modulation by melatonin. Exp Gerontol. 2012;47(9):706-11.
Cardinali DP, Pagano ES, Scacchi Bernasconi PA, et al. Melatonin and mitochondrial dysfunction in the central nervous system. Horm Behav. 2013;63(2):322-30.
Jimenez-Aranda A, Fernandez-Vazquez G, Mohammad ASM, et al. Melatonin improves mitochondrial function in inguinal white adipose tissue of Zucker diabetic fatty rats. J Pineal Res. 2014;57(1):103-9.
Agil A, El-Hammadi M, Jimenez-Aranda A, et al. Melatonin reduces hepatic mitochondrial dysfunction in diabetic obese rats. J Pineal Res. 2015;59(1):70-9.
Baburina Y, Odinokova I, Azarashvili T, et al. 2’,3’-Cyclic nucleotide 3’-phosphodiesterase as a messenger of protection of the mitochondrial function during melatonin treatment in aging. Biochim Biophys Acta.2017;1859(1):94-103.
Stacchiotti A, Favero G, Lavazza A, et al. Hepatic Macrosteatosis Is Partially Converted to Microsteatosis by Melatonin Supplementation in ob/ob Mice Non-Alcoholic Fatty Liver Disease. PLoS One.2016;11(1):e0148115.
Jenwitheesuk A, Nopparat C, Mukda S, et al. Melatonin regulates aging and neurodegeneration through energy metabolism, epigenetics, autophagy and circadian rhythm pathways. Int J Mol Sci.2014;15(9):16848-84.
Teran R, Bonilla E, Medina-Leendertz S, et al. The life span of Drosophila melanogaster is affected by melatonin and thioctic acid. Invest Clin. 2012;53(3): 250-61.
Chang HM, Wu UI, Lan CT. Melatonin preserves longevity protein (sirtuin 1) expression in the hippocampus of total sleep-deprived rats. J Pineal Res. 2009;47(3):211-20.
Rodriguez MI, Escames G, Lopez LC, et al. Improved mitochondrial function and increased life span after chronic melatonin treatment in senescent prone mice. Exp Gerontol. 2008;43(8):749-56.
Anisimov VN, Popovich IG, Zabezhinski MA, et al. Melatonin as antioxidant, geroprotector and anticarcinogen. Biochim Biophys Acta. 2006;1757(5-6): 573-89.
Hevia D, Gonzalez-Menendez P, Quiros-Gonzalez I, et al. Melatonin uptake through glucose transporters: a new target for melatonin inhibition of cancer. J Pineal Res. 2015;58(2):234-50.
Magnanou E, Attia J, Fons R, et al. The timing of the shrew: continuous melatonin treatment maintains youthful rhythmic activity in aging Crocidura russula. PLoS One. 2009;4(6):e5904.
Jackson EK, Menshikova EV, Mi Z, et al. Renal 2’,3’-Cyclic Nucleotide 3’-Phosphodiesterase Is an Important Determinant of AKI Severity after Ischemia-Reperfusion. J Am Soc Nephrol. 2016;27(7):2069-81.
Baburina Y, Azarashvili T, Grachev D, et al. Mitochondrial 2’, 3’-cyclic nucleotide 3’-phosphodiesterase (CNP) interacts with mPTP modulators and functional complexes (I-V) coupled with release of apoptotic factors. Neurochem Int. 2015;90:46-55.
Krestinina O, Azarashvili T, Baburina Y, et al. In aging, the vulnerability of rat brain mitochondria is enhanced due to reduced level of 2’,3’-cyclic nucleotide-3’-phosphodiesterase (CNP) and subsequently increased permeability transition in brain mitochondria in old animals. Neurochem Int. 2015;80:41-50.
Ichas F, Mazat JP. From calcium signaling to cell death: two conformations for the mitochondrial permeability transition pore. Switching from low- to high-conductance state. Biochim Biophys Acta. 1998;1366(1-2):33-50.
Lemasters JJ, Nieminen AL, Qian T, et al. The mitochondrial permeability transition in cell death: a common mechanism in necrosis, apoptosis and autophagy. Biochim Biophys Acta. 1998;1366(1-2):177-96.
Baines CP. The cardiac mitochondrion: nexus of stress. Annu Rev Physiol. 2010;72:61-80.
Bopassa JC, Michel P, Gateau-Roesch O, et al. Low-pressure reperfusion alters mitochondrial permeability transition. Am J Physiol Heart Circ Physiol. 2005;288(6):H2750-5.
Fiskum G. Mitochondrial participation in ischemic and traumatic neural cell death. J Neurotrauma.2000;17(10): 843-55.
Honda HM, Ping P. Mitochondrial permeability transition in cardiac cell injury and death. Cardiovasc Drugs Ther. 2006;20(6):425-32.
Schinder AF, Olson EC, Spitzer NC, et al. Mitochondrial dysfunction is a primary event in glutamate neurotoxicity. J Neurosci. 1996;16(19):6125-33.
Paradies G, Petrosillo G, Paradies V, et al. Melatonin, cardiolipin and mitochondrial bioenergetics in health and disease.J Pineal Res. 2010;48(4): 97-310.
1 note
·
View note
Text
Quercetin protects the vascular endothelium against iron overload damages.
PMID: Eur J Pharmacol. 2020 Feb 5 ;868:172885. Epub 2019 Dec 20. PMID: 31870832 Abstract Title: Quercetin protects the vascular endothelium against iron overload damages via ROS/ADMA/DDAHⅡ/eNOS/NO pathway. Abstract: The aberrant accumulation of iron causes vascular endothelium damage, which is thought to be associated with excess reactive oxygen species (ROS) generation. Quercetin (Que), as a flavonoid, has a certain ability to scavenge free radicals. Therefore, we aimed to explore the protective mechanism of Que on iron overload induced HUVECs injury focused on ROS/ADMA/DDAHⅡ/eNOS/NO pathway. In this study, HUVECs was treated with 50 μM iron dextran and 20 μM Que for 48 h. We found that Que attenuated the damages induced by iron, as evidenced by decreased ROS generation, increased DDAHⅡexpression and activity, reduced ADMA level, increased NO content and p-eNOS/eNOS ratio, and eventually caused a decrease in apoptosis. After addition of pAD/DDAHⅡ-shRNA, the effects of Que mentioned above were reversed. Meanwhile, iron overload induced mitochondrial oxidative stress, reduced mitochondrial membrane potential and increased mitochondrial permeability transition pores (mPTP) opening, which were also partially alleviated by Que. In addition, L-arginine (L-Arg), a ADMA competition substrate, ciclosporin A (CsA), a mPTP blocking agent, and edaravone (Eda), a free radical scavenger, were used as positive control reagents. The effects of Que were similar to that of L-Arg, CsA and Eda treatment. These results illustrated that Que could attenuate iron overload induced HUVECs mitochondrial dysfunction via ROS/ADMA/DDAHⅡ/eNOS/NO pathway.
read more
0 notes
Text
The Connection Between Damaged Mitochondria and Arthritis
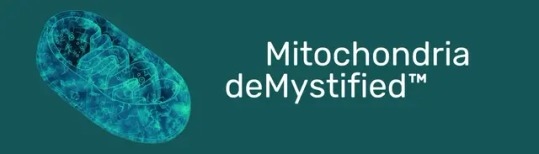
Mitochondria are integral organelles responsible for various critical cellular functions, primarily energy production through oxidative phosphorylation. They are involved in maintaining cellular homeostasis, regulating metabolism, modulating calcium levels, and controlling apoptosis. Emerging evidence has highlighted mitochondrial dysfunction as a key contributor to a variety of diseases, including arthritis. This formal overview aims to explore the complex relationship between damaged mitochondria and arthritis, focusing on the molecular mechanisms that link mitochondrial dysfunction to the pathogenesis of inflammatory joint diseases, particularly rheumatoid arthritis (RA) and osteoarthritis (OA).
Mitochondrial Structure and Function
Mitochondria are double-membraned organelles found in eukaryotic cells, and they are crucial for cellular energy metabolism. Their primary role is the production of adenosine triphosphate (ATP) via oxidative phosphorylation, a process that takes place in the inner mitochondrial membrane. During this process, the electron transport chain (ETC) generates a proton gradient across the inner membrane, which drives ATP synthesis through ATP synthase. However, this process also generates reactive oxygen species (ROS) as byproducts, primarily from complexes I and III of the ETC. Under normal physiological conditions, ROS are neutralized by antioxidants, including superoxide dismutase (SOD), catalase, and glutathione. However, under pathological conditions, excessive ROS production can lead to oxidative stress, contributing to cellular damage and dysfunction.
In addition to ATP production, mitochondria have essential roles in calcium buffering, apoptosis regulation, and the maintenance of cellular integrity. Damage to these organelles disrupts these functions, contributing to various diseases, including arthritis.
Mitochondrial Dysfunction in Arthritis
Arthritis is a group of diseases characterized by inflammation and degeneration of the joints. It includes conditions like rheumatoid arthritis (RA), an autoimmune disease, and osteoarthritis (OA), a degenerative disease. In both types of arthritis, mitochondrial dysfunction has been identified as a critical factor that exacerbates disease progression through several mechanisms, including increased oxidative stress, immune activation, and tissue damage.
1. Oxidative Stress and Mitochondrial Damage
Oxidative stress is a hallmark of both RA and OA, and mitochondria are central to its production. In these conditions, mitochondrial dysfunction results in an increase in ROS production, overwhelming the cell’s antioxidant defenses. This oxidative stress leads to the modification of cellular structures, including proteins, lipids, and DNA, causing further mitochondrial damage. In RA, pro-inflammatory cytokines such as tumor necrosis factor-alpha (TNF-α), interleukin-1 (IL-1), and interleukin-6 (IL-6) stimulate immune cells like macrophages and neutrophils to release large amounts of ROS. These ROS contribute to the local inflammatory environment and accelerate joint destruction by damaging mitochondria and amplifying oxidative stress.
Mitochondrial damage results in a feedback loop where impaired mitochondrial function generates more ROS, further promoting inflammation. For instance, in RA, markers of oxidative damage such as 8-hydroxy-2'-deoxyguanosine (8-OHdG) and malondialdehyde (MDA) have been found to correlate with disease activity, suggesting a direct relationship between mitochondrial dysfunction and disease severity.
2. Mitochondrial DNA Damage and Inflammatory Signaling
Mitochondrial DNA (mtDNA) is particularly vulnerable to oxidative damage due to its proximity to the ETC, where ROS are produced during ATP synthesis. Unlike nuclear DNA, mtDNA is not protected by histones and has limited repair mechanisms, making it prone to mutations. Damage to mtDNA impairs mitochondrial function and can lead to the release of mtDNA fragments into the cytoplasm or extracellular space.
In the context of arthritis, mtDNA damage has been implicated in immune activation. When damaged mtDNA is released into the cytoplasm, it is recognized by pattern recognition receptors (PRRs), such as toll-like receptors (TLRs), on immune cells. TLRs, particularly TLR9, activate downstream inflammatory signaling pathways that lead to the production of pro-inflammatory cytokines such as TNF-α and IL-6. This further exacerbates the inflammatory response in joints and contributes to the progression of arthritis. Studies have shown that the presence of mtDNA fragments in the serum of RA patients correlates with disease activity, indicating the role of mtDNA in driving inflammation.
3. Mitochondrial Dynamics and Arthritis Pathogenesis
Mitochondrial dynamics refer to the continuous processes of mitochondrial fission (division) and fusion (joining), which maintain mitochondrial function and integrity. Fission allows for the removal of damaged mitochondria, while fusion helps to integrate mitochondrial contents and maintain a healthy mitochondrial pool. Imbalance between fission and fusion is associated with several diseases, including arthritis.
In the case of RA, excessive mitochondrial fission and reduced fusion have been observed. This imbalance results in mitochondrial fragmentation, which impairs mitochondrial function, increases ROS production, and contributes to cellular stress. Fission is regulated by proteins such as dynamin-related protein 1 (Drp1) and fission 1 protein (Fis1), while fusion is controlled by mitofusins (Mfn1 and Mfn2) and optic atrophy 1 (OPA1). Dysregulation of these proteins in RA leads to a fragmented mitochondrial network, which exacerbates oxidative stress and inflammation in synovial tissues.
4. Mitochondrial-Dependent Cell Death
Mitochondria are also central regulators of programmed cell death, particularly apoptosis. In the pathogenesis of arthritis, excessive or dysregulated apoptosis contributes to joint destruction. Mitochondrial dysfunction plays a critical role in the intrinsic apoptotic pathway by releasing pro-apoptotic factors such as cytochrome c and apoptosis-inducing factor (AIF). These factors activate caspase-dependent and caspase-independent pathways, leading to the death of synovial cells and cartilage cells, which contributes to the progressive tissue damage observed in both RA and OA.
Furthermore, mitochondrial permeability transition pore (mPTP) opening, which is induced by oxidative stress, can lead to necrosis, a form of uncontrolled cell death. Necrotic cell death in the joints increases inflammation and tissue degradation, particularly in OA, where cartilage breakdown is a hallmark feature.
Therapeutic Approaches Targeting Mitochondrial Dysfunction in Arthritis
Given the significant role of mitochondrial dysfunction in the pathogenesis of arthritis, various therapeutic strategies aimed at improving mitochondrial function are under investigation.
1. Mitochondrial Antioxidants
Mitochondrial-targeted antioxidants, such as MitoQ and MitoTEMPO, have been developed to selectively accumulate in mitochondria, where they can neutralize ROS and reduce oxidative stress. These compounds have shown promise in preclinical models of arthritis, where they help to reduce inflammation, protect mitochondrial function, and limit joint damage. The use of mitochondrial antioxidants could be an effective strategy to mitigate oxidative stress in arthritic conditions.
2. Mitochondrial Biogenesis Enhancement
Another potential therapeutic approach is the activation of mitochondrial biogenesis, the process by which new mitochondria are formed to compensate for damaged mitochondria. Agents that activate peroxisome proliferator-activated receptor gamma coactivator 1-alpha (PGC-1α), a key regulator of mitochondrial biogenesis, could help restore mitochondrial function in arthritic tissues. Compounds such as resveratrol and NAD+ precursors are under investigation for their ability to promote mitochondrial biogenesis and improve cellular metabolism in arthritis.
3. Mitochondrial Dynamics Modulation
Restoring the balance between mitochondrial fission and fusion is another therapeutic strategy. Inhibiting excessive mitochondrial fission or promoting mitochondrial fusion may help maintain mitochondrial integrity and reduce inflammation in arthritis. Drugs targeting Drp1 or enhancing Mfn1/Mfn2 activity are potential candidates for modulating mitochondrial dynamics in arthritic diseases.
4. Mitophagy Enhancement
Mitophagy, the selective autophagic degradation of damaged mitochondria, is essential for maintaining mitochondrial quality. Enhancing mitophagy through the use of compounds like spermidine or activators of the PINK1/PARK2 pathway could help eliminate dysfunctional mitochondria and reduce inflammation, making it a promising therapeutic approach in arthritis.
Conclusion
Mitochondrial dysfunction plays a critical role in the pathogenesis of arthritis, contributing to oxidative stress, inflammation, and joint damage. The intricate relationship between damaged mitochondria and immune activation highlights the importance of targeting mitochondrial health in the treatment of arthritis. Emerging therapeutic strategies aimed at restoring mitochondrial function, reducing oxidative stress, and modulating mitochondrial dynamics hold promise for improving the management of arthritis and preventing joint destruction. Further research into mitochondrial biology and its role in arthritis is essential for the development of more effective, targeted therapies for these debilitating conditions.
#Mitochondrial dysfunction#Autoimmune disorders#Oxidative stress#Reactive oxygen species (ROS)#Mitochondrial dynamics#Mitochondrial fission#Mitochondrial fusion#Mitophagy#Apoptosis#Mitochondrial DNA (mtDNA)#Damage-associated molecular patterns (DAMPs)#Immune cell activation#Systemic lupus erythematosus (SLE)#Rheumatoid arthritis (RA)#Multiple sclerosis (MS)#Pattern recognition receptors (PRRs)#Toll-like receptors (TLRs)#Pro-inflammatory cytokines#Cytochrome c#NF-κB signaling#MitoQ#MitoTEMPO#Spermidine#PINK1/PARK2 pathway#Mitochondrial-targeted antioxidants#Immune dysregulation#Chronic inflammation#Mitochondrial fragmentation#Mitochondrial permeability transition pore (mPTP)#Autoantibodies
0 notes
Text
Current and upcoming mitochondrial targets for cancer therapy
Abstract
Mitochondria are essential intracellular organelles that regulate energy metabolism, cell death, and signaling pathways that are important for cell proliferation and differentiation. Therefore, mitochondria are fundamentally implicated in cancer biology, including initiation, growth, metastasis, relapse, and acquired drug resistance. Based on these implications, mitochondria have been proposed as a major therapeutic target for cancer treatment. In addition to classical view of mitochondria in cancer biology, recent studies found novel pathophysiological roles of mitochondria in cancer. In this review, we introduce recent concepts of mitochondrial roles in cancer biology including mitochondrial DNA mutation and epigenetic modulation, energy metabolism reprogramming, mitochondrial channels, involvement in metastasis and drug resistance, and cancer stem cells. We also discuss the role of mitochondria in emerging cancer therapeutic strategies, especially cancer immunotherapy and CRISPR-Cas9 system gene therapy.
Abbreviations
ETC, electron transport complexes;
ROS/RNS, reactive oxygen or nitrogen spices;
∆ψm, mitochondrial inner membrane potential;
TCA, TriCarboxylic Acid Cycle;
MPTP, mitochondrial permeability transition pore;
mtDNA, mitochondrial DNA;
ND5, NADH dehydrogenase 5;
mtDNMT1, mtDNA methyltransferase;
SAM, S-adenosyl-L-methionine;
IDH, Isocitrate dehydrogenase;
SDH, succinate dehydrogenase;
FH, fumarate hydratase;
GSH, glutathione;
HIF-1α, hypoxia-inducible transcription factor-1 α;
KEAP1, Kelch-like ECH-associated protein 1;
NRF2, nuclear farter (erythroid-derived 2)-like 2;
PDC, pyruvate dehydrogenase complex;
PDHE1α, PDC E1α subunit;
PDK1, 2, 3 and 4, pyruvate dehydrogenase kinase 1, 2, 3 or 4;
PDP1 and 2, pyruvate dehydrogenase phosphatases 1 or 2;
VDAC, voltage dependent anion channel;
ANT, adenine nucleotide translocator;
CypD, cyclophilin D;
MAC, mitochondrial apoptosis-induced channel;
mitoKv1.3, mitochondrial voltage-gated potassium channel;
MitoIKCa/KCa3.1, mitochondrial intermediated conductance calcium activated potassium channel;
BKCa/KCa1.1, large conductance calcium activated potassium channel;
SKCa/KCa3.1, small conductance calcium activated potassium channel;
mitoKATP, Mitochondrial ATP-sensitive potassium channel;
TASK-3, TWIK-related Acid-sensitive K+ channel-3;
KCNK9 UCP, uncoupling proteins;
MCU, Mitochondria Ca2+ uniporter;
TNBC, triple-negative breast cancer;
Mrs2, mitochondrial Mg2 + channel;
TOM/TIM, translocase of outer/inner membrane;
PGC1-α, peroxisome proliferator-activated receptor gamma, coactivator 1 alpha;
CSC, cancer stem cell;
PRX3, peroxiredoxin 3;
PD-1, programmed death-1;
CTLA-4, cytotoxic T lymphocyte antigen 4;
LAG-3, lymphocyte activation gene 3;
Tim-3, T cell immunoglobulin and mucin-containing gene 3;
CRISPR–Cas9, clustered regularly interspaced short palindromic repeats–CRISPR-associated 9;
CLIC4, chloride intracellular channel 4
Keywords
mitochondria;
mitochondria channels;
cancer stem cell;
cancer immunotherapy;
CRISPR-Cas9
Corresponding author at: National Research Laboratory for Mitochondrial Signaling, Department of Physiology, Cardiovascular and Metabolic Disease Center, College of Medicine, Inje University, Bokji-ro 75, Busanjin-gu, Busan 47392, Republic of Korea.
© 2017 Published by Elsevier Ltd.
Note to users: Accepted manuscripts are Articles in Press that have been peer reviewed and accepted for publication by the Editorial Board of this publication. They have not yet been copy edited and/or formatted in the publication house style, and may not yet have the full ScienceDirect functionality, e.g., supplementary files may still need to be added, links to references may not resolve yet etc. The text could still change before final publication.
Although accepted manuscripts do not have all bibliographic details available yet, they can already be cited using the year of online publication and the DOI, as follows: author(s), article title, Publication (year), DOI. Please consult the journal's reference style for the exact appearance of these elements, abbreviation of journal names and use of punctuation.
When the final article is assigned to volumes/issues of the Publication, the Article in Press version will be removed and the final version will appear in the associated published volumes/issues of the Publication. The date the article was first made available online will be carried over.
Author : Hyoung Kyu Kim, Yeon Hee Noh, Bernd Nilius, Kyung Soo Ko, Byoung Doo Rhee, Nari Kim, Jin Han
— Seminars in Cancer Biology
0 notes
Text
Mitochondrial Dysfunction and the Pathogenesis of Alzheimer’s Disease
Introduction
Mitochondria are pivotal organelles responsible for adenosine triphosphate (ATP) production via oxidative phosphorylation (OXPHOS), regulation of calcium homeostasis, reactive oxygen species (ROS) generation, and the initiation of apoptotic pathways. The brain’s high energy demands and sensitivity to oxidative stress make mitochondrial functionality essential for neuronal health. Recent findings underscore the critical role of mitochondrial dysfunction in the molecular mechanisms driving Alzheimer’s disease (AD), a progressive neurodegenerative disorder characterized by memory loss and cognitive decline. This article provides an in-depth exploration of how mitochondrial impairment contributes to AD pathogenesis and examines emerging therapeutic approaches.
Alzheimer’s Disease: Pathological Hallmarks
Alzheimer’s disease is the most prevalent form of dementia and presents neuropathological features including extracellular amyloid-beta (Aβ) plaques, intracellular neurofibrillary tangles (NFTs) of hyperphosphorylated tau, synaptic dysfunction, and neuronal death. Despite decades of research, the precise etiology remains complex, involving genetic predispositions, environmental triggers, and metabolic imbalances. Mitochondrial dysfunction emerges as a central mechanism intertwining these multifactorial contributors.
Mitochondrial Functions in Neurons
Neurons depend on mitochondria for sustaining synaptic transmission, neurotransmitter synthesis, and maintaining ionic gradients essential for action potentials. Beyond energy production, mitochondria modulate intracellular calcium dynamics, synaptic plasticity, and apoptotic signaling, all of which are integral to learning and memory. Impaired mitochondrial function disrupts these processes, resulting in neuronal vulnerability and degeneration.
Mechanisms of Mitochondrial Dysfunction in Alzheimer’s Disease
Oxidative Stress and ROS Dysregulation: Mitochondria are the primary source of ROS, which, under physiological conditions, participate in cellular signaling. In AD, impaired OXPHOS elevates ROS production, leading to oxidative damage of mitochondrial DNA (mtDNA), proteins, and lipids. This oxidative stress contributes to synaptic deficits, neuronal death, and exacerbates Aβ aggregation and tau hyperphosphorylation.
Amyloid-Beta-Mediated Mitochondrial Toxicity: Aβ peptides localize within mitochondria, where they interact with the inner mitochondrial membrane and components of the electron transport chain (ETC). This interaction inhibits ATP synthesis, augments ROS generation, and compromises mitochondrial membrane potential. Additionally, Aβ disrupts axonal transport of mitochondria, depriving synaptic regions of necessary energy supply.
Tau Pathology and Mitochondrial Dysfunction: Hyperphosphorylated tau impairs microtubule stability, disrupting the intracellular trafficking of mitochondria and other organelles. Dysregulated tau also affects mitochondrial dynamics, including fission and fusion processes, leading to an accumulation of damaged and fragmented mitochondria.
Calcium Homeostasis Impairment: Mitochondria act as buffers for cytosolic calcium. In AD, dysregulated calcium signaling exacerbates mitochondrial calcium overload, triggering permeability transition pore (mPTP) opening and initiating apoptotic cascades. Calcium dysregulation also potentiates Aβ aggregation and tau phosphorylation.
Defects in Mitochondrial Dynamics and Mitophagy: Proper mitochondrial function relies on a balance between fission and fusion processes. In AD, this balance is disrupted, leading to mitochondrial fragmentation and a reduction in mitochondrial network integrity. Impaired mitophagy—the selective autophagic clearance of damaged mitochondria—further exacerbates mitochondrial dysfunction and neuronal degeneration.
Genetic Correlations Between Mitochondrial Dysfunction and Alzheimer’s Disease
APOE-ε4 Allele: The APOE-ε4 variant, a significant genetic risk factor for sporadic AD, has been associated with heightened oxidative stress and impaired mitochondrial efficiency.
Presenilin Mutations: Mutations in PSEN1 and PSEN2, linked to familial AD, disrupt calcium signaling and mitochondrial function, exacerbating cellular stress and neuronal loss.
mtDNA Mutations: Increased somatic mutations and deletions in mtDNA observed in AD patients suggest a direct mitochondrial genomic contribution to the disease.
Therapeutic Strategies Targeting Mitochondrial Dysfunction
Antioxidant Therapies:
Mitochondria-targeted antioxidants such as coenzyme Q10, MitoQ, and SS-31 aim to mitigate oxidative stress and preserve mitochondrial integrity.
Enhancement of Mitochondrial Biogenesis:
Pharmacological agents activating PGC-1α (peroxisome proliferator-activated receptor gamma coactivator-1α) enhance mitochondrial biogenesis, improving neuronal energy metabolism.
Calcium Modulation:
Drugs like memantine, an NMDA receptor antagonist, help restore calcium homeostasis, reducing excitotoxicity and mitochondrial stress.
Promotion of Mitophagy:
Urolithin A and other mitophagy-enhancing compounds facilitate the clearance of defective mitochondria, preventing their accumulation and associated toxicity.
Gene-Based Therapies:
Gene-editing technologies such as CRISPR/Cas9 offer potential for correcting mtDNA mutations and modulating genes implicated in mitochondrial quality control.
Lifestyle Interventions:
Dietary Approaches: Ketogenic diets and caloric restriction enhance mitochondrial efficiency and reduce ROS.
Physical Exercise: Regular aerobic activity stimulates mitochondrial biogenesis and improves oxidative resilience.
Optimized Sleep: Adequate sleep promotes mitochondrial repair and the removal of toxic protein aggregates such as Aβ.
Advancements and Research Directions
Emerging research employs cutting-edge technologies like single-cell transcriptomics, super-resolution microscopy, and metabolomics to unravel the mitochondrial mechanisms underlying AD. Novel drug delivery systems targeting mitochondria and the development of nanotechnologies further hold promise for precision therapeutics.
Conclusion
Mitochondrial dysfunction is a cornerstone in the complex pathogenesis of Alzheimer’s disease, intersecting with oxidative stress, Aβ and tau pathology, calcium dysregulation, and impaired dynamics. Targeting mitochondrial pathways through pharmacological interventions, gene therapy, and lifestyle modifications offers a promising avenue for mitigating disease progression. Continued research into mitochondrial biology and its interplay with neurodegeneration is essential for developing transformative therapies for Alzheimer’s disease.
#Mitochondrial dysfunction#Alzheimer’s disease#Neurodegeneration#Oxidative stress#Reactive oxygen species (ROS)#Amyloid-beta (Aβ)#Tau pathology#Calcium homeostasis#Mitochondrial dynamics#Mitophagy#Electron transport chain (ETC)#Mitochondrial biogenesis#ATP production#Apoptosis#APOE-ε4 allele#Presenilin mutations#mtDNA mutations#Synaptic dysfunction#Neurofibrillary tangles (NFTs)#Antioxidant therapies#Gene-based therapies#Lifestyle interventions#Ketogenic diets#Caloric restriction#Physical exercise#Precision therapeutics
0 notes
Text
Nobiletin could alleviate iron overload damage in vascular endothelium.
PMID: Biol Trace Elem Res. 2020 Jan 30. Epub 2020 Jan 30. PMID: 32002792 Abstract Title: Nobiletin Regulates ROS/ADMA/DDAHII/eNOS/NO Pathway and Alleviates Vascular Endothelium Injury by Iron Overload. Abstract: Iron overload is harmful to health and associates with intracellular excessive reactive oxygen species (ROS) generation. Nobiletin (Nob) is known to be antioxidant and anti-inflammatory. However, whether Nob can protect endothelial cells against iron overload has not been studied, and the specific mechanism has not yet been elucidated. In this study, we have identified the protective effects of Nob, and its underlying molecular mechanism in human umbilical vein endothelial cells (HUVECs) suffered from iron overload via ROS/ADMA/DDAHII/eNOS/NO pathway. We found that compared with 50 μM iron dextran treatment, co-treatment with 20 μM Nob increased cell viability and decreased lactate dehydrogenase activity. Besides, Nob could upregulate DDAHII expression and activity, promote eNOS phosphorylation to produce more NO, reduce ADMA content, and therefore increase superoxide dismutase, catalase, and glutathione peroxidase activities, and decrease malondialdehyde level and ROS generation. Nob also inhibited mitochondrial permeability transition pore (mPTP) openness and cleaved caspase-3 expression, and decreased apoptosis induced by iron overload. These results were consistent when Nob was replaced by the positive control reagents L-arginine (a competitive substrate of ADMA), cyclosporin A (an mPTP closing agent), or edaravone (a free radical scavenger). The addition of pAD/DDAHII-shRNA adenovirus reversed the above effects of Nob. These data suggested that the protective mechanism of Nob was to inhibit ROS burst, upregulate DDAHII expression and activity, promote eNOS phosphorylation, produce NO, reduce ADMA content, and ultimately alleviate iron overload damage in vascular endothelium.
read more
0 notes
Text
Lycopene protects against myocardial ischemia-reperfusion injury.
PMID: Drug Des Devel Ther. 2019 ;13:2331-2342. Epub 2019 Jul 11. PMID: 31371925 Abstract Title: Lycopene protects against myocardial ischemia-reperfusion injury by inhibiting mitochondrial permeability transition pore opening. Abstract: Background: Mitochondria permeability transition pore (MPTP) is an important therapeutic target for myocardial ischemia-reperfusion injury (MIRI). Lycopene (LP) is a potent antioxidant extracted from the mature fruits of plants and has been reported to protect against MIRI; however, its mechanism of action has yet to be completely elucidated. The present study aimed to investigate the role of MPTP in the cardioprotection of LP.Methods: H9c2 cells were pretreated with LP for 12 hrs and were subjected to 12-hr hypoxia/1-hr re-oxygenation, and cell viability was measured by a Cell Counting Kit-8 (CCK-8) assay. Male rats were subsequently intraperitoneally injected with LP for 5 consecutive days. At 24 hrs following the final injection, the rat hearts were isolated and subjected to 30-min ischemia/120-min reperfusion using Langendorff apparatus. The myocardial infarct size was measured by a TTC stain. Opening of the MPTP was induced by CaCland measured by colorimetry. The change in mitochondrial transmembrane potential (ΔΨm) was observed under a fluorescence microscope. Apoptosis was measured by flow cytometry and a TUNEL stain, and the expression of apoptosis-related proteins was detected by Western blotting.Results: LP pretreatment significantly increased cell viability, reduced myocardial infarct size and decreased the apoptosis rate. In addition, opening and the decrease ofΔΨm were attenuated by LP and the expressions of cytochrome, APAF-1, cleaved caspase-9 and cleaved caspase-3 were also decreased by LP. However, these beneficial effects on MIRI were abrogated by the MPTP opener (atractyloside). Furthermore, LP treatment markedly increased Bcl-2 expression, decreased Bax expression and the Bax/Bcl-2 ratio.Conclusion: The results of the present study demonstrated that LP protects against MIRI by inhibiting MPTP opening, partly through the modulation of Bax and Bcl-2.
read more
0 notes