#corona study
Explore tagged Tumblr posts
Text
you know, i suppose you could say that tlt has a love triangle

like, that's a claim you can make

but i just think

that that would be

oversimplifying

just a little bit

#these look so shitty but i made this in 20 minutes as a study break#the locked tomb#my post#also that line between alecto and corona should be reversed#it's supposed to reference nona's crush on corona
449 notes
·
View notes
Text
thinking thoughts about how nona was so obsessed with crown, and crown specifically- not coronabeth. crown, with her boots and her cargo pants and her guns and her hair tied back, with all her charm and strength, all her rage and determination.
was that really just nona? or, walk with me here- is there a chance that that was actually alecto, too, bleeding through and rising to the surface?
alecto, seeing a kind of kinship in crown- in this big, tall, strong blonde with a sword strapped to her back, hot and lovely and kind and awful and powerful and perfect. this woman who refuses to give up- on her sister, on saving jody, on BOE's resistance. who's unafraid to throw one hell of a tantrum, if it means being listened to, for once. crown, who everyone thinks of as dumb, who everyone underestimates, who no one ever takes as seriously as they should, even though she's clearly capable of plenty of atrocities in her own right. this woman who's been described over and over again as someone who positively radiates life, and energy, and vitality, and strength. this woman who wanted nothing more than the chance to be herself, to be free, to serve as cavalier and guardian and protector, but was instead sentenced at birth to a life of being a princess and wearing dresses and looking pretty and loving less and staying out of the way and keeping her mouth shut and playing second fiddle to a necromancer obsessed with power and glory. familiar, no? this woman who was betrayed, left behind, left alone, and left utterly in the dark by the one person who's supposed to love her the most- only to then be told that being abandoned was in her best interest, really, for her own safety.
thinking about all the times we've seen ianthe insult crown's intelligence and praise her beauty in the same breath. you big dumb bimbo, what can you do? of all the times we've seen ianthe fussing over crown's appearance. thinking of the sister-lyctor makeover-montage ahead of dios apate minor, and how harrow hated every second of it, and how ianthe treated it like nostalgic second nature. thinking about the third house: fucked-up planet gossip-girl with all its betrayal and espionage and flesh magic and debauchery, three for the gleam of a jewel or a smile. thinking about the pressure that must have come with keeping up the double-necromancer ruse, about ianthe having successfully played the part of two necromancers from the age of six. exactly how much practice must that have taken? thinking about the casual, automatic, possessive, offhanded, violating nature of ianthe playing god and giving harrow a full head of fast-growing hair without asking, without even telling her, just to make harrow prettier, just to piss her off, just because she could. how she did it so easily, and without hesitation, almost as though she's maybe done that sort of thing before.
thinking about preservation. about a perfect body frozen in ice for a myriad, about ianthe spending all her downtime on the mithraeum figuring out how long she can keep an apple core in perfect stasis before the rot sets in.
thinking about corpse puppeting: a deceased world leader here, a trusted cavalier and friend you've known from the cradle there. about i picked you to change, and this is how you repay me? about she took babs. and who even cares about babs? babs! she could have taken me!
thinking about alecto, and hollywood hair barbie, and you have made me a hideousness.
thinking about crown, who's by her own admission boobs and hair and talk and a hell of a swordhand.
thinking about something as simple as stud earrings, and about how much grief ianthe gave her for daring to wear them.
nona loved crown.
something tells me that alecto might, too.
#the locked tomb#alecto the ninth#alecto#coronabeth tridentarius#crown him with many crowns#ianthe tridentarius#locked tomb meta#am i tagging this right? idk#nona the ninth spoilers#harrow the ninth spoilers#to be PERFECTLY clear i love ianthe. and i still think it's ENTIRELY possible that corona will turn out to be Badtwin in the end#but truly who's to say. women's wrongs and whatnot#also ik nona WAS alecto but like. she was also her own person. To Me. a study in 'would i be a different person without all the baggage' et#tridentarii#what a fascinating fucked up little dynamic with those two
509 notes
·
View notes
Text
This is all about Jote now
#Ffxvi modern au#Got stuck trying to think what would Joshua be in a modern au#Historian? A teacher? Would he study law? Then figure it would be really funny if he is a writer and is with a pharmacist#Also with Tarja on quick dial#Not in my cannon! Jote going to have friends in this au and she is buddy with Tarja now#Joshua getting corona like more than once is canon and you know it#You know Clive was calling constantly and radiating nerves energy because of it all through quarantine#Dear Jill has to deal with it all#Clive having the biggest softest spot for Jote after meeting her the first time#Actually Joshua Jote mid and torgal could get Clive to do anything#She would be the type to over work herself and Prince Charming would have to step in#Jote#joshua rosfield#ffxvi#final fantasy xvi#clive rosfield#Tarja
368 notes
·
View notes
Note
if you got to live in one of the seven *cough cough eight cough* kingdoms, which would it be?
options:
- corona
- galcrest
- ingvarr
- pittsford
- koto
- neserdnia
- bayangor
- selene/sceptra/dark kingdom
hmmmm
i mean
somehow corona seems the most functional from what i remember?
which is honestly. such a low bar.
but uhh
yeah i mean like
dark kingdom pre evil moonstone stuff
otherwise
i guess
corona
#marble asks#i would kill/die for (almost) every member of the brotherhood bc im normal like that#but also im a small boy and am incapable of fighting#(for real i can barely handle the like 10 seconds of stage combat i have in tlt)#id be one of the generic people in corona#id be like#so useless except for the one filler episode where raps or another mc needs something really specific#like information about foxglove toxins or studies done on the intelegence of rooks#/silly
7 notes
·
View notes
Text
Can someone please tell me to go do my homework? I am. Procrastinating.
#If there is anything i found out abt myself during my time in school and especially during corona lockdown#Is that i simply Won't do stuff without peer pressure#And what did college give me? No peers.#I am not in the environment i am meant to be in....i miss having mentors and study circles....uuuuuu
4 notes
·
View notes
Text

👑 Queen Arianna and her sister, Willow 🌸🎨
#Queen Arianna#Willow#Tangled the Series#Rapunzel’s Tangled Adventure#The Way of Willow#character study#Disney fanart#late night drawing#sisters#Kingdom of Corona#Tangled#Rapunzel#King Frederic#sisterly love#Julie Bowen#Jane Krakowski#Mandy Moore#Disney Channel#Disney Channel cartoons
4 notes
·
View notes
Text
raps - centric
☼ ┊ headcanon & meta ┊ ❛ she's a girl with the best intentions ❜ ☼ ┊ visage ┊ ❛ i have magic hair that glows when i sing ❜ ☼ ┊ character study ┊ ❛ who's afraid of little old me? ❜ ☼ ┊ aesthetic ┊ ❛ the floating lanterns gleam ❜ ☼ ┊ wardrobe ┊ ❛ sew a dress! ❜ ☼ ┊ desires ┊ ❛ in the heat of your electric touch ❜ ☼ ┊ older visage ┊ ❛ queen of corona ❜
#☼ ┊ headcanon & meta ┊ ❛ she's a girl with the best intentions ❜#☼ ┊ visage ┊ ❛ i have magic hair that glows when i sing ❜#☼ ┊ character study ┊ ❛ who's afraid of little old me? ❜#☼ ┊ aesthetic ┊ ❛ the floating lanterns gleam ❜#☼ ┊ wardrobe ┊ ❛ sew a dress! ❜#☼ ┊ desires ┊ ❛ in the heat of your electric touch ❜#☼ ┊ older visage ┊ ❛ queen of corona ❜
0 notes
Text

The Corona Paradox - Solving Important Physical Problems 2024
The founder of the Suns Water and Global Greening Organization has started some new and really great projects in 2024. These include new books and publications such as the scientific article on the coronal heating paradox and possible solutions to many of the known problems with very logical and evidential solutions. The new book and the key study on the Sun's Water Theory have helped to solve many problems almost completely. During the month, further studies supported the ongoing work and a new book and research project on solar science and physics was started. The new book, entitled “The Corona Paradox”, will be published by summer next year. This pre-publication is based on one of the drafts without further corrections. Constructive feedback and support is always welcome. https://sunswater.org/
#solar study#suns water#sun theory#solar theory#academic#artistic#artwork#atmosphere#corona#coronal#discovery#education#energy#forces#gravity#heating#innovation#paper#physics#physical#research#solar energy#solar science#solar#study#sun#sunlight#water
0 notes
Text
The Corona Paradox - Solving Important Physical Problems 2024
This is a comprehensive and detailed summary of the scientific article and short study about one of the biggest unsolved physical problems. It is an extract and preview for the final publication. It is also an introduction for a new book with focus on scientific issues related to solar science.
Solving Problems of Solar Physics: Evidence, Key Factors and Mechanisms
The coronal heating paradox refers to the fundamental mystery of why the Sun's corona, the outermost layer of the solar atmosphere, is vastly hotter than the photosphere beneath it. Despite being farther from the Sun's energy-producing core, the corona reaches temperatures of 1-3 million Kelvin, whereas the photosphere is a relatively cool and can heat up to 5,500°C (5,800 K). The scientific paradox challenges traditional models of solar energy transport and has led to intense research into the underlying mechanisms that explain the temperature difference. Recent developments in solar physics, including studies of magnetic field dynamics, wave-particle interactions, and particle acceleration, have provided comprehensive solutions to this puzzle.
During studies for the Sun’s Water Theory the lead researcher discovered many solution approaches to known problems of solar science. He found many crucial factors and answers to resolve the coronal paradox almost completely – together with known and unknown solutions. It was one of the most perplexing problems in solar physics, first identified in the 1940s, where observations show that the temperature of the Sun's outer atmosphere is much higher than its visible surface or photosphere with an average temperature of around 5,500 K. The temperature discrepancy has been a longstanding mystery with the central question: How can the Sun's corona be so much hotter than the photosphere? The main question is answered and many of the problems are solved in this scientific article and in other sections of the special Suns Water study project with further research. It follows a comprehenisve overview of main factors that can drive coronal heating.
1. Alfvén Waves and Their Role in Heating Processes
Alfvén waves are primary candidates for transporting energy from the Sun’s interior to the corona. These magnetohydrodynamic (MHD) waves propagate along magnetic field lines in plasma. The dispersion relation for Alfvén waves is: ω² = k²v_A², where ω is the wave frequency, k is the wave number, and v_A is the Alfvén speed (v_A = B/√(μ₀ρ)), which depends on the magnetic field strength B, plasma density ρ, and permeability of free space μ₀. These waves are widely discussed as contributors to coronal heating. They transport both energy and momentum, and when dissipated, transfer energy to the plasma, causing heating. A key feature of Alfvén waves in coronal heating is their ability to carry energy from the solar surface (photosphere) to the corona. The dissipation of Alfvén waves involves resonant absorption, where wave energy is absorbed by plasma at specific frequencies. This process depends on magnetic field geometry, plasma density, and wave frequency.
A modified model for resonant absorption can be written as: dE/dt = (B² / μ₀) * [ν_r / (1 + ν_r²)] , where dE/dt is the rate of energy absorption, B is the magnetic field strength, μ₀ is the permeability of free space, and ν_r is the wave frequency relative to the plasma’s resonant frequency. For temperature variations, the speed is modified as: v_A = B / √(μ₀ρ) * (1 + δT) , where δ is a constant and T is the temperature (K). This modification accounts for the temperature dependence of wave propagation. In high-temperature plasmas, resistivity changes influence wave dissipation rates. Temperature fluctuations, such as during solar flares and coronal mass ejections (CMEs), can enhance wave heating. Observations from Hinode and SDO have detected wave modes in coronal loops, supporting resonant absorption with frequencies that match theoretical predictions. Studies of the Sun’s Water Theory will refine these calculations, aiding the understanding of solar processes.
A further modification for wave energy dissipation is: v_A = (B + E_w) / √(μ₀ρ) , where E_w is the energy contribution from Alfvén waves. This adjustment reflects the significant role of wave dissipation in heating the corona. Alfvén wave energy interacts with plasma, potentially causing nonlinear wave processes that deposit energy into the plasma. Wave damping occurs through mechanisms like resonant absorption and mode conversion. Understanding how energy is transferred and dissipated is crucial. Turbulent cascading in the corona can enhance wave dissipation. As small-scale turbulence develops, Alfvén waves transfer energy across scales, converting it into heat. This process may explain the high temperatures in the corona relative to the photosphere, providing continuous energy input to the coronal plasma. More details on Alfvén waves and heating mechanisms can be found in other sections.
Observational and Empirical Evidence: The role of Alfvén waves in coronal heating has been examined using high-resolution instruments like Hinode, SDO, and TRACE. Observations of coronal loops and active regions have revealed oscillations matching the signatures of Alfvén waves. Specifically, SDO's Atmospheric Imaging Assembly (AIA) detected high-frequency oscillations in coronal loops, with periods corresponding to Alfvén wave frequencies. These waves are believed to propagate upward from the photosphere, carrying energy to the corona. Hinode's EUV Imaging Spectrometer (EIS) has observed resonant absorption in coronal loops. Studies of quasi-periodic pulsations (QPPs) in flare regions also support the presence of Alfvén waves and their role in energy dissipation. Furthermore, coronal seismology with SDO data has confirmed that observed oscillations align with theoretical Alfvén wave predictions. These waves contribute to localized heating and are thought to play a major role in the high temperatures observed in the corona.
2. Bow Shocks and Bernoulli’s Equation
Bernoulli's equation and bow shocks provide insights into the coronal heating paradox, especially regarding energy transfer, plasma dynamics, and solar wind acceleration. While they don't fully resolve the paradox, these concepts shed light on specific processes related to the heating and acceleration of the solar corona. Bow Shocks and Energy Dissipation Bow shocks occur when the solar wind interacts with surrounding media, such as the Earth's magnetosphere or interstellar space. While typically associated with planetary magnetospheres, the concept also helps understand shock heating in the Sun's corona, particularly in active regions and where the solar wind interacts with the Sun’s magnetic fields. In the corona, shock waves, generated by reconnection or plasma flows, can dissipate energy as magnetic fields reorganize. These shocks convert the kinetic energy of plasma into thermal energy, contributing to coronal heating.
Shock Heating and Plasma Dynamics: When the solar wind encounters denser or slower-moving regions (e.g., near active regions or coronal loops), bow shocks compress and heat the plasma. The interaction between fast solar wind and slower coronal material creates a shock front, dissipating energy as heat, which increases the temperature of the corona. Shock Heating Equation: ΔT = (γ - 1) * (P2 - P1) / ρ , where ΔT is the temperature increase across the shock, γ is the adiabatic index (specific heat ratio), P2 and P1 are the pressures before and after the shock, and ρ is the plasma density. This equation shows how the shock dissipates the kinetic energy of solar wind particles, converting it into heat, potentially explaining the temperature differences observed in the corona.
Bernoulli’s equation relates the pressure, velocity, and potential energy of a fluid in steady flow. Although it is primarily used for incompressible fluids, its principles can be adapted to the solar corona to understand the energy balance between coronal heating and solar wind acceleration. The equation is expressed as: P + 0.5 * ρ * v² + ρ * g * h = constant , where P is pressure, ρ is plasma density, v is plasma velocity, g is gravitational acceleration, and h is height. In the corona, this equation helps link the pressure and velocity of the solar wind, illustrating the connection between coronal heating and solar wind acceleration. As plasma in the corona heats, it expands and accelerates, moving from high-pressure, high-temperature regions to lower-pressure areas in the outer corona and solar wind.
Bernoulli’s Energy Balance in the Solar Wind: ΔP = 0.5 * ρ * (v2² – v1²) , where ΔP is the pressure difference between two points, ρ is plasma density, and v2 and v1 are the velocities at two different points in the corona or solar wind. This equation shows that an increase in velocity leads to a pressure decrease, which aligns with observations that solar wind accelerates as it moves away from the Sun’s corona. The relationship between thermal, kinetic, and magnetic energy in the corona may influence coronal heating, providing insight into energy transfer mechanisms.
Pressure-Velocity Relationship in the Corona: The dissipation of magnetic energy (e.g., through magnetic reconnection) could increase the corona's pressure. According to Bernoulli’s principle, this could cause a corresponding increase in plasma velocity. The accelerated plasma then carries energy away from the corona, contributing to both coronal heating and the outward flow of solar wind. In regions with higher solar wind speed, Bernoulli’s equation suggests that thermal energy is converted into kinetic energy, driving solar wind acceleration. Therefore, magnetic energy conversion to heat in the corona may also drive solar wind acceleration, linking heating and wind processes.
Bow shocks and Bernoulli’s equation together offer explanations for solar wind acceleration. Both mechanisms transfer energy from thermal or magnetic forms into kinetic energy as plasma moves outward. Bow shocks dissipate energy from turbulent or fast solar wind, increasing plasma temperature in localized regions. In areas where the solar wind speed is low and coronal pressure is high, Bernoulli’s equation suggests thermal energy conversion into kinetic energy, driving solar wind acceleration. Therefore, energy conversion at shock fronts and velocity transitions may be responsible for both coronal heating and solar wind acceleration.
Coronal Heating through Shock-Driven Waves: Shock-driven waves, generated by turbulence or reconnection events, could propagate through the corona, similar to how bow shocks dissipate energy in planetary magnetospheres. These waves could contribute to coronal heating by converting kinetic energy into thermal energy. The propagation of waves (e.g., Alfvén waves) through the corona leads to energy dissipation when they encounter plasma regions with varying densities or magnetic fields, creating localized heating. These shock- and wave-driven mechanisms might help explain how small-scale processes like nanoflares or magnetic reconnection sustain the high temperature in the solar atmosphere, even though the source of this heat remains unclear.
While bow shocks and Bernoulli’s equation do not provide definitive solutions to the coronal heating paradox, they offer valuable insights, especially regarding energy transfer and plasma dynamics in the corona and solar wind. These concepts help explain how thermal energy is converted to kinetic energy, contributing to solar wind acceleration, and how magnetic energy dissipation might lead to localized coronal heating. Further research into how these mechanisms interact with other processes, such as wave dissipation and turbulence, will deepen our understanding of coronal heating.
3. Chemical Processes and Element Abundance
The Sun's corona, primarily composed of plasma, plays a crucial role in energy transport and magnetic structure formation, which influence heating processes. While the coronal heating paradox is often framed in terms of physical mechanisms such as waves, reconnection, and turbulence, chemical processes and element abundances are also vital factors in determining the observed temperature distribution in the corona. The chemical composition, dominated by hydrogen and helium, includes trace amounts of heavier elements such as oxygen, carbon, neon, iron, and silicon. The interactions between these elements significantly influence both heating and cooling rates in the corona.
Hydrogen is the most abundant element in the solar wind and corona. It primarily exists as protons (H⁺), which are crucial in maintaining the ionization equilibrium, especially at temperatures exceeding 1 million K. Proton interactions with the surrounding plasma through Coulomb collisions with electrons and heavier ions facilitate energy transfer, thereby heating the electron population, which in turn heats the ions. Proton-ion and proton-electron collisions contribute to energy dissipation and radiative losses via Bremsstrahlung. The low ionization potential of hydrogen (~13.6 eV) makes it easily ionizable in the corona, and as a result, H⁺ ions participate significantly in energy absorption during magnetic reconnection events and wave-particle interactions.
Contributions of Specific Elements to Coronal Heating: Carbon (C), especially in its C⁴⁺ state, plays an important role in coronal heating. C⁴⁺ ions are highly effective at absorbing UV radiation, thus maintaining the ionization balance in the corona. Carbon ions contribute to radiative cooling through emission lines in the UV and X-ray ranges, particularly the carbon Ly-α line. As the corona's temperature increases, carbon ions transition through various ionization states, which significantly impacts the thermal equilibrium and energy transport in the corona. Their interaction with the plasma through electron-ion collisions also contributes to energy dissipation.
Helium (He), primarily in the forms of He⁺ and He²⁺, contributes to the radiative cooling of the corona. Although less abundant than hydrogen, helium's role remains significant due to its emission and absorption processes. The He²⁺ ion, with a higher ionization energy, interacts with high-energy photons and charged particles, particularly during magnetic field interactions and wave heating. These interactions lead to the emission of UV radiation, which plays a role in the overall cooling of the corona. Helium's interactions with the magnetic field are also crucial in regulating the ionization balance of the plasma.
Iron (Fe), one of the most chemically significant elements in the solar corona, exists in highly ionized states (Fe⁶⁺, Fe⁷⁺, Fe⁸⁺, etc.). Iron ions are vital for both the absorption and emission of radiation. Fe⁶⁺ ions, in particular, absorb high-energy UV and X-ray radiation and re-emit energy in the form of X-ray lines, contributing to significant radiative losses. These ions are found in the hottest regions of the corona, where they also contribute to Bremsstrahlung radiation, further enhancing energy dissipation. The high charge states of iron ions allow them to interact significantly with both electromagnetic radiation and magnetic fields, especially during solar flares or coronal mass ejections (CMEs).
Iron's Role in Magnetic Reconnection and Wave Heating: Iron ions, especially Fe⁶⁺ and Fe⁷⁺, interact with magnetic fields and Alfvén waves in the corona. These ions efficiently absorb energy from MHD waves and magnetic reconnection processes, which leads to ion acceleration, increased ion temperature, and subsequent heating of the plasma. During solar flares or CMEs, these interactions can contribute to magnetic energy dissipation and ion heating, thereby enhancing the coronal heating process.
Magnesium (Mg), primarily in the form of Mg⁶⁺ and Mg⁷⁺ ions, contributes to energy dissipation through collisional ionization and Bremsstrahlung processes. Although less abundant than iron or oxygen, magnesium plays an important role in wave-particle interactions, especially at higher temperatures. Neon (Ne), in the form of Ne⁶⁺ and Ne⁷⁺, also plays a role in coronal heating by absorbing UV radiation and emitting X-rays. Neon’s ability to maintain a high ionization state at the temperatures present in the corona makes it an efficient contributor to the thermal balance of the plasma.
Nitrogen (N): Although less abundant, nitrogen, especially in its N⁴⁺ state, plays a role in radiative cooling. It absorbs high-energy radiation and emits UV and X-rays when excited by energetic events such as solar flares or magnetic reconnection. While its contribution to the overall coronal heating process is smaller than other elements like carbon or oxygen, nitrogen still impacts the energy dynamics, particularly in the transition region between the photosphere and the corona.
Oxygen (O) is one of the most important elements in the solar corona, primarily in the form of O⁶⁺ and O⁷⁺ ions. Oxygen ions are abundant in the transition region and upper corona, where they absorb and emit radiation in the UV and X-ray spectra. O⁶⁺ ions, in particular, absorb significant amounts of solar radiation, and undergo recombination and excitation processes that contribute to radiative losses. Oxygen also plays a role in energy dissipation through Bremsstrahlung and collisional ionization, with O⁶⁺ ions being highly sensitive to changes in electron density and temperature, providing critical information about coronal heating mechanisms.
Silicon (Si), in its highly ionized states (Si⁶⁺ and Si⁷⁺), contributes to both radiative cooling and ionization balance in the corona. Like iron, silicon emits radiation in the X-ray range and absorbs energy from magnetic fields and waves, releasing it as thermal radiation. Silicon ions contribute to the overall heating processes by interacting with energetic solar events, such as flares or CMEs, and dissipating energy into the surrounding plasma.
Collisional Excitation and De-excitation: Collisions between electrons and ions lead to the excitation of ions to higher energy states, and as the ions relax to lower energy states, they emit photons. This process, known as collisional de-excitation, can be described by the rate: R_exc = n_e * n_i * σ_exc * v_rel , where σ_exc is the excitation cross-section, and v_rel is the relative velocity between electrons and ions. The emitted photons, observed as spectral lines in the UV and X-ray ranges, provide essential diagnostic information about the temperature and density of the plasma. These emission lines are crucial for diagnosing energy transfer and dissipation mechanisms in the corona. The rate of collisional ionization can be expressed as: R_ci = n_e * n_i * σ_ci * v_rel , where σ_ci is the collisional ionization cross-section, and this process helps maintain the ionization state in the corona, especially in hotter regions.
Ionization and Recombination Processes: Ionization rates are crucial for understanding the dynamics of energy transfer in the corona. The ionization of elements like hydrogen, oxygen, and iron influences plasma conductivity and the overall energy balance. The Saha equation is often used to model ionization equilibria in stellar atmospheres: (n_i / n_e) = (2 * π * m_e * k_B * T)^(3/2) * (g_i / g_e) * exp(-I_i / k_B T) , where n_i is the ion density, n_e the electron density, T is temperature, and I_i is the ionization energy. The balance between ionization and recombination rates dictates the ionization state of the plasma, influencing coronal heating processes. Recombination, the reverse of ionization, occurs when a free electron combines with an ion to form a neutral species. The recombination rate is the inverse of the ionization rate, and the rate of recombination is given by: R_recomb = n_e * n_i * β(T) , where β(T) is the recombination coefficient, dependent on the plasma temperature. The interplay between ionization and recombination rates is essential for maintaining the ionization balance, and thus for controlling the energy dynamics in the corona.
Line Emission and Ionized Metals: Ionized metals like Fe, O, C, and Si contribute to coronal heating through line emissions, which provide diagnostic information about the plasma's temperature and ionization state. These emissions arise from collisional excitation followed by de-excitation and are observed in solar spectroscopy. The line intensity, given by: L_line = n_e * n_i * σ_exc * h * ν , where h is Planck's constant and ν is the frequency of the emitted radiation, helps determine the plasma's thermal state and is key to understanding energy dissipation in the corona.
High-Temperature Chemistry: The ionization states of elements like iron, magnesium, and silicon can affect the thermal conductivity of the plasma and influence wave energy dissipation. The ionization and recombination processes are temperature-dependent, and they modify the plasma's heat capacity. The overall state of the plasma is described by the equation of state (EOS), which links temperature, density,
4. Convection and Energy Transport in the Photosphere
Convection is a crucial mechanism of energy transport in the Sun’s convective zone, just below the photosphere. In this zone, thermal convection dominates: hot plasma rises, cools as it nears the surface, and sinks again. This convective energy transport takes over when radiation alone is no longer sufficient to transfer energy through the Sun's outer layers.
Convective Energy Transport (Adiabatic Process): At around 0.7 solar radii, the Sun’s temperature drops to about 2 million K, and the plasma becomes opaque to radiation. This is when convection becomes the primary energy transport mechanism. The convective zone extends from 0.7 to 1.0 solar radii and involves the rise of hot plasma and sinking of cooler plasma, akin to a boiling pot of water. In this region, convection becomes dominant because conduction is inefficient due to the opacity of the material.
The convective heat flux is given by the equation: Q = (dT/dz) * c_p * ρ * v , where dT/dz is the temperature gradient, c_p the specific heat capacity at constant pressure, ρ the plasma density and v is the convective velocity. This equation shows how the temperature gradient drives the convection process and transports energy from the deeper layers of the Sun to the photosphere. The convective motion is driven by buoyancy: when plasma at lower levels heats up, it becomes less dense and rises. Upon reaching the surface, it cools, becomes denser, and sinks, continuing this cycle. The convection process forms granules and supergranules on the Sun's surface, these small convection cells (~1000 km in size) are visible in solar observations. The convection cells are caused by the decreasing temperature with altitude, which triggers hot material to rise and cooler material to sink, creating a dynamic convection pattern.
Convective Velocity and Granulation: The Rayleigh number helps describe the transition from stable conduction to turbulent convection. When Ra exceeds a certain threshold, convection becomes turbulent, resulting in the formation of large convection cells. The granules seen on the Sun’s surface are part of these cells. The Rayleigh number is given by: Ra = (g * β * ΔT * L³) / (ν * α) , where g is the gravitational acceleration, β the thermal expansion coefficient, ΔT the temperature difference, L the characteristic length scale (the convective cell size), ν the kinematic viscosity and α is the thermal diffusivity. Once the energy reaches the photosphere, it is radiated as electromagnetic radiation, including visible light and ultraviolet radiation. The photosphere marks the point where the plasma becomes transparent enough for light to escape. The temperature here is approximately 5,500 K, and photons can travel freely from this layer into space.
5. Energy Conservation and Dissipation
Understanding the energy conservation and dissipation mechanisms in the corona is key to addressing the coronal heating paradox. Despite the corona being much hotter than the photosphere, traditional energy transport mechanisms like radiation and conduction cannot fully explain this temperature difference. A comprehensive analysis of energy conservation and dissipation is therefore necessary.
Cooling by Radiation: A major cooling mechanism in the corona is Bremsstrahlung radiation, which occurs when charged particles, such as electrons, are decelerated or accelerated in the presence of other charged particles (ions). The cooling rate due to Bremsstrahlung is given by: L = n_e² Λ(T) , where n_e is the electron number density and Λ(T) is the radiative loss function, which depends on the temperature of the plasma. This form of cooling is most significant in the lower corona, where the plasma density is higher and radiation losses are more prominent.
Dissipation of Waves in the Corona: Alfvén waves are a key mechanism for transporting energy from the photosphere to the corona. These waves must dissipate their energy to heat the plasma in the corona. This can happen via several mechanisms, including mode conversion (where Alfvén waves turn into magnetoacoustic waves) and resonant absorption (where the wave energy is absorbed by the plasma). The heating rate from wave dissipation can be expressed as: P_wave = α W , where α is the wave dissipation efficiency (a dimensionless constant) and W is the energy carried by the wave. Once the wave energy is dissipated, it is transferred to the plasma, heating it.
Energy Balance Equation for the Corona: In a steady-state system, the energy balance equation for the solar corona can be written as: (∂E/∂t) + ∇ * F = Q - L , where E is the total energy density, F the energy flux, Q the heating rate (which includes mechanisms like wave dissipation and magnetic reconnection) and L is the cooling rate (due to radiation and conduction). This equation captures how energy is transported, supplied by heating mechanisms, and lost through cooling processes.
Energy Flux and Heat Conduction: The energy flux is described by Fourier's Law: F = -κ ∇T , where: κ is the thermal conductivity and ∇T is the temperature gradient.
In the solar corona, the Spitzer conductivity is used to model heat transport: κ = 1.84 × 10⁻⁵ T^(5/2) / n_e , where T is the temperature and n_e is the electron number density. In the presence of magnetic fields, thermal conduction is suppressed perpendicular to the field lines. The effective conductivity in the corona is much lower than the Spitzer value for an unmagnetized plasma. This reduced thermal conductivity may contribute to the build-up of heat in magnetic loops, helping to explain why the corona is hotter than the photosphere.
Heat Loss via Expansion (Adiabatic Cooling): Another important cooling mechanism in the corona is adiabatic cooling. As plasma moves outward in the solar wind, its density decreases, causing an expansion that leads to cooling. This cooling becomes more significant at higher altitudes, where plasma expands more rapidly. The cooling rate due to expansion is: L_expansion = n_e T / (γ - 1) ∇v , where γ is the adiabatic index (about 5/3 for a monoatomic ideal gas) and v is the plasma velocity.
Refined Energy Balance for the Corona: While the basic energy balance equation captures the general idea of heating and cooling in the corona, a more refined model must consider complex processes like turbulent energy cascades, resistive dissipation, and wave-particle interactions. A more detailed energy balance equation that includes these dissipative processes is: (∂E/∂t) + ∇ * (κ ∇T) = P_source - P_loss , where P_source is the heating rate from mechanisms such as wave dissipation, magnetic reconnection, and non-thermal particle acceleration. P_loss is the cooling rate, which accounts for radiative cooling, adiabatic cooling, turbulent dissipation, and resistive effects. This refined equation demonstrates that energy is not only transported via conduction but is also generated by heating mechanisms and lost through various cooling processes. Improving our understanding of energy input (such as wave dissipation) and refining the loss mechanisms (such as turbulent dissipation) will help explain the high temperatures observed in the corona.
Energy Losses and Cooling Mechanisms: One of the challenges in the coronal heating paradox is understanding how energy is stored and dissipated in the corona despite substantial energy losses. Radiation losses, especially in regions with high density and temperature, are dominated by optically thin emission processes from ionized ions like Fe IX-XIII. The radiative cooling rate can be expressed as: Q_rad = n_e * n_i * Λ(T) , where n_e is the electron density, n_i is the ion density and (T) is the radiative cooling function. In the corona, the presence of strong magnetic fields limits the efficiency of thermal conduction perpendicular to the field lines. The Spitzer conductivity for an unmagnetized plasma can be used. However, in the corona, the effective conductivity is much lower due to the magnetic fields, which hinder the flow of heat perpendicular to the magnetic field lines. This lower thermal conductivity suggests that heat cannot efficiently escape along magnetic loops, allowing thermal energy to build up, thus explaining the higher temperatures in the corona compared to the photosphere.
6. Energy Transport in the Sun’s Layers
Energy transport in the Sun's layers varies significantly with depth and plasma conditions. In the photosphere, radiation dominates, although convective upwelling creates granulation - regions of rising hot plasma surrounded by cooler sinking material. This convection pattern visibly transports energy at the Sun's surface. In the outer layers (chromosphere and corona) energy transport becomes more complex. The chromosphere still involves radiation but is more transparent at lower temperatures. In the corona, energy is primarily transported via magnetic reconnection, Alfvén waves, and turbulent processes. This increase in temperature with height is central to the coronal heating paradox, where mechanisms like wave dissipation and magnetic dynamics help explain the high corona temperatures. The Sun’s energy transport processes differ by region: radiation dominates in the core and radiative zone, where photons slowly diffuse outward. Convection takes over in the outer layers, rapidly moving energy to the surface. The photosphere emits visible light, while the chromosphere and corona involve complex wave and magnetic processes, which are vital for understanding phenomena like solar flares, sunspots, and the solar wind.
Energy Balance in the Photosphere: The Sun's photosphere energy balance links core energy production with the sunlight emitted. Energy generated by nuclear fusion in the core travels outward and is emitted as electromagnetic radiation at the photosphere. This balance helps explain the Sun’s internal energy generation and the coronal heating paradox.
Energy Generation in the Core (Hydrogen Fusion): The Sun’s energy comes from nuclear fusion in its core, where hydrogen nuclei fuse to form helium, releasing energy in the form of gamma rays and neutrinos. This energy is transported outward, eventually emitted as sunlight. The total luminosity of the Sun corresponds to the energy generated by fusion. The energy generation rate per unit mass describes the energy produced per kilogram of the Sun's core per second. The core's luminosity: L_core = ε * m_core , with ε as the energy generation rate (W/kg) and m_core as the core mass (kg), can be calculated and estimated. For the Sun, ε ≈ 2.5 × 10⁶ W/kg, this value sets the baseline for the energy reaching the photosphere, though it takes thousands to millions of years for energy to diffuse outward.
Steady-State Energy Balance: In a steady state, the energy radiated from the photosphere matches the energy generated in the core. The luminosity is given by: L = 4πR²σT_ph⁴ , where L is the luminosity, R is the Sun's radius, σ is the Stefan-Boltzmann constant, and T_ph is the photosphere’s temperature. This equation shows that luminosity depends on the surface area of the photosphere and its temperature. The photosphere radiates all energy generated by fusion and even by energetic processes and forces we don’t know or still not understand.
8. Heat Transfer in the Photosphere
The heat transfer in the photosphere is a delicate balance between radiation and convection. While radiation dominates the outward transfer of energy from the core, the convective zone just beneath the photosphere plays a significant role in shaping the Sun’s surface structure and appearance. The temperature gradient across these layers is crucial for understanding how energy moves through the Sun’s outer layers and contributes to phenomena like the solar granulation observed at the photosphere.
Convective Heat Transfer (Adiabatic Process): In the convective zone just beneath the photosphere, heat is transported by convection. The heat flux due to convection can be approximated by the following formula (for a convective layer): Q = ρ * c_p * v * ΔT , where Q is the heat flux (W/m²), ρ the density of the plasma (kg/m³), c_p the specific heat capacity of the plasma (J/kg·K), v the convective velocity (m/s) and ΔT is the temperature difference between the convective material and the surrounding area (K). In the photosphere, the convective heat transfer is responsible for the appearance of granulation patterns, where hot gas rises to the surface and cooler material sinks. The convective process is responsible for the granulation patterns visible on the Sun’s surface, where hot gas rises to the surface, loses heat, and sinks. These patterns are a direct result of the convective cells at the photosphere. The granules have characteristic sizes of a few thousand kilometers, and their motions indicate a rapid heat transfer from the deeper layers to the surface. Convective heat transfer affects mostly the Sun's surface layers. Beyond the photosphere the behavior of energy becomes less straightforward, especially in the corona where radiative transfer and magnetic interactions dominate.
Temperature Gradient and Convective Instability: The temperature gradient in the convective zone is steep, with a drop of approximately 2 million K in the outermost part of the Sun's interior to around 5,500 K at the photosphere. The convective instability in this region is driven by the Boussinesq approximation to hydrodynamics, where the buoyancy force is the driving factor behind convection. The resulting convective cells transport heat more efficiently than pure radiation alone could, but the efficiency of this process decreases as the material becomes more transparent to radiation in the outer layers. Despite the efficiency of convection in the convective zone, the mechanism that transfers heat from the photosphere into the corona remains a central challenge.
9. Kinetic Theory of Plasma and Particle Interactions
The kinetic theory of plasma provides a more detailed, microscopic understanding of the behavior of plasma compared to fluid models. In the context of coronal heating, the kinetic theory is vital for explaining the fine-scale dynamics of particle interactions, energy transport, and dissipation processes in the corona. Fluid models often assume a continuum approach, where the plasma is treated as a smooth fluid, but this overlooks the discrete interactions between individual particles. The kinetic theory, on the other hand, explicitly accounts for these interactions and is essential for understanding phenomena like wave-particle interactions, collisions, and particle acceleration in the corona.
Boltzmann Transport Equation and Distribution Function: The key equation that governs the dynamics of particle distribution in a plasma is the Boltzmann transport equation. This equation describes how the distribution function of particles f(v, r, t) evolves over time under the influence of external forces and internal collisions. The equation is given by: ∂f / ∂t + v * ∇f + (F / m) * ∇_v f = C[f] , where f(v, r, t) is the distribution function, which gives the number density of particles at a given velocity v, position r, and time t. ∇f is the gradient of the distribution function in real space (m⁻¹) and (F / m) represents the force per unit mass (N/kg), with F as external force (e.g., electromagnetic force). ∇_v f is the gradient in velocity space, representing how the distribution of particles changes with respect to their velocities. C[f] is the collision term, which represents the rate of change of the distribution function due to collisions between particles or interactions between particles and waves. The collision term is crucial in the context of the coronal heating paradox because it governs the rate of energy dissipation in the plasma. In the corona, the collision frequency is generally low, but wave-particle interactions (through Alfvén waves, ion-cyclotron waves, etc.) can significantly affect the energy distribution, leading to particle heating and wave damping. These processes contribute to the overall coronal heating mechanism.
10. Magnetic Reconnection and Energy Release
Magnetic reconnection is a key mechanism in heating the solar corona. During reconnection events, oppositely directed magnetic fields reconnect, releasing energy in the form of thermal and kinetic energy, and accelerated particles. The Sweet-Parker model estimates the reconnection speed in a collisional plasma: v_A = v_ideal * (L / d) , where v_ideal is the Alfvén velocity, L the reconnection region’s scale, d the current sheet thickness, and ρ is the plasma density. The velocity (v_A = B / √(μ₀ρ) relates to the magnetic field strength and plasma density. Reconnection in the corona results in high temperatures, contributing to phenomena like solar flares. However, the Sweet-Parker model underestimates reconnection speed in high-energy environments, prompting the development of alternative models.
Modification with Lundquist number S: Faster reconnection at higher Lundquist numbers is a potential solution to coronal heating, with the dissipated magnetic energy heating the plasma. Numerical simulations, incorporating multi-fluid and kinetic effects, help improve understanding of these complex processes.
Observations of Solar Flares: Data from instruments like GOES and SDO show rapid energy release during solar flares, consistent with reconnection models. These processes correlate with specific X-ray and EUV emissions, providing direct evidence of heating.
Petschek Reconnection Model: Unlike the Sweet-Parker model, the Petschek model predicts faster reconnection due to shock-like structures forming at the ends of the current sheet. The reconnection speed in the Petschek model is: V_rec ∝ V_A / ln(L / δ) , where δ is the ion diffusion region, and L is the reconnection region length. This model suggests faster reconnection speeds and more efficient energy release, enhancing coronal heating. Stronger magnetic fields and lower plasma densities accelerate reconnection.
11. Nanoflares and Magnetic Reconnection
Nanoflares are small bursts of energy that heat plasma in the corona to millions of Kelvin. They often coincide with high-energy electron populations, which are accelerated during magnetic reconnection. These electrons deposit their energy into the surrounding plasma, contributing to coronal heating.
Energy Dissipation during Reconnection: Energy released in magnetic reconnection is mainly thermal, heating the plasma. The total power dissipated during reconnection is: P_reconnect = η J² V , where η is plasma resistivity, J is current density, and V is the reconnection region volume. This dissipation is crucial in solar flares and other active regions, driving coronal heating.
Energy Release during Magnetic Reconnection: Magnetic reconnection occurs at various scales, releasing energy through heat and high-energy particles. The energy released is proportional to current density, magnetic field strength, and reconnection speed: E_reconnect = η * (J * B) * V , where η is the resistivity of the plasma, J the current density, B the magnetic field strength and V is the reconnection velocity. Magnetic reconnection remains one of the key drivers of coronal heating. This process leads to the formation of hot plasma regions in which ions are rapidly heated. During solar flares, magnetic reconnection releases vast amounts of energy, accelerating particles and ionizing species like Fe⁶⁺ and O⁶⁺ to high temperatures. These energetic particles are trapped in magnetic loops, where they interact with the plasma, causing further ionization and contributing to radiative cooling. Magnetic reconnection also plays a major role in the acceleration of Coronal Mass Ejections (CMEs), which are massive bursts of solar wind carrying charged particles from the Sun’s corona. These ejections can lead to enhanced ionization in the corona as the released energy heats the surrounding plasma. The amount of energy released during these processes can be quantified using models of magnetic flux, such as the Sweet-Parker model or the Petschek model of reconnection. The energy conversion efficiency is directly related to the ionization state of the plasma and the density of charged particles like Fe⁶⁺ and O⁶⁺. Read more about solar flares and CMEs in the Sun’s Water study and research papers for solar science.
Nanoflare Statistics and Energy Contribution: Although individual nanoflares release small amounts of energy, their cumulative effect can significantly contribute to maintaining coronal temperatures. Statistical analyses and numerical simulations suggest that nanoflares, occurring on a sub-minute timescale, could release energies ranging from 10⁸ to 10¹² erg, enough to sustain the corona’s high temperature. Data from instruments like Hinode and the Parker Solar Probe support this idea.
Non-Thermal Electron Heating: Non-thermal electrons, accelerated by magnetic reconnection or wave-particle interactions, play a major role in coronal heating. These electrons transfer energy to the plasma via collisions and wave-particle interactions, contributing to the coronal heating paradox by raising the corona’s temperature without direct heating from the photosphere.
Electron Energy Distribution: The energy distribution of accelerated electrons follows a power-law: f(E) ∝ E^−α , where α typically ranges from 1.5 to 2.0 for flare-associated electrons. The rate of energy deposition depends on electron density and collision rates with the ambient plasma: Pe = ne * σe * ve * ΔE , where ne is the electron density, σe the electron collision cross-section, ve the electron velocity and ΔE is the energy deposited in the plasma.Nanoflares, with their small-scale energy bursts, contribute to coronal heating through electron acceleration and energy deposition.
Shock Acceleration: In solar flares and CMEs, shock waves accelerate particles to high energies. These shocks, driven by reconnection and other dynamic processes, lead to a power-law distribution of particle energies: f(E) = f_0 * (E / E_0)^(−α) , where E is the energy, f_0 is a normalization constant, and α is the power-law index.
Stochastic Heating: In this process, electrons gain energy from turbulent magnetic fluctuations caused by reconnection. The Kolmogorov turbulence model describes how energy cascades from large to small scales, heating particles in the process. The energy distribution of electrons in a turbulent field is given by: f(E) ∝ E^(-γ) , where γ depends on turbulence level.
12. Non-Linear MHD Turbulence and Wave Dissipation
Magnetohydrodynamic (MHD) turbulence is central to the theory of coronal heating. It involves chaotic, non-linear interactions between magnetic fields and plasma, leading to the dissipation of wave energy as heat. MHD turbulence follows a power-law distribution, where energy cascades from large to small scales, eventually dissipating as heat. The energy density at a wavenumber k is: E(k) = C * k^(-5/3) , where C is a constant. This turbulence, observed in both the solar wind and the corona, is crucial for heating the plasma.
Dissipation of Alfvén Waves in the Corona: Alfvén waves, which propagate from the solar surface into the corona, dissipate their energy through non-linear wave damping or resonant absorption. This process is influenced by plasma density and magnetic field configuration. Resonant absorption occurs when waves become resonant with plasma at specific heights, transferring energy from the wave to the plasma.
Wave Damping by Resonance: The rate of wave energy dissipation in a resonant plasma is given by: γ = (ω² * δ) / (B²) , where γ is the damping rate, ω is the wave frequency, δ is a factor related to plasma density and magnetic field strength, and B is the magnetic field strength. This dissipation increases plasma temperature by converting wave energy into thermal energy.
Observational Evidence: Turbulence leads to current sheets and high-frequency waves, which dissipate energy as heat. Data from SOHO and SDO show significant turbulence in the corona, with wave-breaking and current sheet formation, supporting the idea that turbulence is essential for energy transfer and dissipation in the solar corona.
13. Observational Evidence and Empirical Models
Empirical data from solar missions, including Hinode, IRIS, SDO, and SOHO, have clarified the role of various heating mechanisms in the corona. More details on these missions are provided in the following sections. The integration of key findings, coronal seismology, high-resolution imaging, and spectroscopic data has enabled the refinement of theoretical models of coronal heating. Observations of solar flares, magnetic reconnection, wave propagation, and particle acceleration provide direct evidence that these processes contribute to corona heating. High-resolution SDO data have been instrumental in advancing our understanding of coronal heating. The AIA and EVE instruments aboard SDO have captured detailed images of solar flare emissions and the magnetic fields involved. These observations confirm that energy is released and dissipated in the corona through magnetic reconnection, wave-particle interactions, and Alfvén wave dissipation. Similarly, Hinode has provided crucial data on coronal loops, revealing oscillations and magnetic reconnection events contributing to heating. IRIS has delivered spectral data showing energy flow through the transition region between the chromosphere and corona. These insights and observations highlight fast magnetic reconnection events and small-scale plasma heating, reinforcing the idea that local reconnection significantly contributes to coronal heating. In addition to direct observations, numerical simulations based on empirical data have further validated these heating mechanisms. Models simulating wave propagation, magnetic reconnection, and turbulence replicate the observed temperature distribution in the corona, supporting the mechanisms maintaining high temperatures. Detailed research data can be obtained through ESA and NASA's official communication channels.
Empirical observations from solar missions have provided critical insights into spatial and temporal variations in the Sun’s corona, supporting theoretical models of coronal heating. This section summarizes key findings and empirical evidence. These scientific approaches offer solutions to understanding coronal heating and other physical processes. By integrating research papers, references, studies, and key data, many challenges can be addressed. This article and advanced research from the solar science study contribute to developing a unified coronal heating theory, summarizing key mechanisms in the solar atmosphere and photosphere. The author works on a book called "The Corona Paradox", this article contains some parts of the first chapers. The scientific project and work will improve our understanding of the Sun’s inner layers and the heliosphere, particularly regarding sunlight, solar winds, radiation, and particles. These advances will accelerate progress in planetary science, solar research, and space exploration.
Chromospheric and Coronal Coupling in Active Regions: Recent observations from the Atacama Large Millimeter Array (ALMA) and Interface Region Imaging Spectrograph (IRIS) have provided invaluable data linking the chromosphere to the corona. These observations suggest that energy transfer between the chromosphere and corona is critical in resolving the coronal heating paradox. ALMA data show that magnetic fields in active regions are highly dynamic, enabling continuous energy transfer between these layers. Energy is transferred through field-aligned currents, which assist in heat conduction upward, leading to localized heating and potentially micro-flares or small-scale magnetic reconnection events in the corona. IRIS has captured spectral data on chromospheric jets in active regions, showing that these jets channel energy into the corona via magnetic reconnection. The energy transferred from the chromosphere is significant enough to contribute to coronal heating when combined with wave and particle interactions. Chromospheric coupling, therefore, plays a key role in heating the corona. This energy exchange via magnetic reconnection and dynamic plasma flows is essential for maintaining the high temperatures of the solar corona.
Coronal Bright Points and Small-Scale Heating Events: High-resolution imaging of the solar corona has revealed coronal bright points (CBPs), which are small, localized regions of enhanced brightness linked to transient heating events. Observations from Hinode's EUV Imaging Spectrometer (EIS) and SDO's Atmospheric Imaging Assembly (AIA) have identified intense heating in these areas, often associated with small-scale magnetic flux concentrations in the solar atmosphere. These bright points are thought to result from localized magnetic reconnection and current sheet formations, contributing to small-scale or transient heating events. These observations help refine our understanding of how the corona reaches its high temperatures without large-scale or global heating processes. Coronal bright points are primarily driven by localized magnetic reconnection, where oppositely directed magnetic fields release stored energy as heat. Regions with high electrical current density (current sheets) formed during reconnection are a source of Joule heating, contributing to localized temperature increases. Correlations between CBPs and magnetic flux concentrations suggest that small-scale processes, like magnetic reconnection and current sheet formation, are critical in heating the corona. These findings challenge traditional models based on large-scale heating, supporting the idea that localized processes, such as micro-flares and reconnection events, are essential to coronal heating. Observing these small-scale phenomena in high resolution enhances our understanding of fine-scale interactions in the solar atmosphere and their role in the corona’s energy balance.
Coronal Loop Dynamics: Observations of coronal loops reveal complex magnetic structures that likely contribute to localized heating through magnetic reconnection. These loops, often exhibiting temperatures of 1–2 million K, support the hypothesis that reconnection is responsible for heating in these regions. Coronal loops act as energy storage reservoirs, accumulating energy that is later released via magnetic reconnection or wave heating. Observations from the Parker Solar Probe and SDO show that coronal loops can trap hot plasma, storing energy before releasing it. AIA observations have revealed that these loops undergo dramatic temperature fluctuations, with localized heating spikes near their footpoints. The loops focus wave energy from the lower atmosphere, concentrating it at higher altitudes. These oscillations and reconnection events in coronal loops contribute to solving the coronal heating paradox by storing and releasing energy in a controlled manner.
Coronal Mass Ejections (CMEs) and Solar Flares: Solar missions like Hinode, SDO, and SOHO have provided extensive data on solar flares and CMEs. SOHO's LASCO instrument has captured detailed images of CMEs, while SDO's AIA has monitored the solar corona during flare events. These observations reveal dramatic changes in the coronal magnetic field, consistent with the theory that magnetic reconnection releases energy during these events. SDO's EVE has detected large X-ray and ultraviolet emissions from solar flares, directly indicating energy release during magnetic reconnection. High-energy particles accelerated during flares are detected by instruments like ACE, providing evidence of particle acceleration and corona heating. Hinode’s X-ray observations of flare events show localized heating in the corona, with temperatures reaching millions of degrees. These findings confirm that magnetic reconnection releases large amounts of energy into the corona, contributing to both explosive events like CMEs and the steady heating that sustains the corona’s high temperatures.
Coronal Stratification and Magnetic Field Dynamics: The corona’s temperature stratification is influenced by complex interactions between magnetic fields, wave dissipation, and non-radiative heating mechanisms. Energy is transferred from the chromosphere to the higher layers of the corona, where magnetic fields become more dynamic. In these regions, energy is deposited through wave dissipation, magnetic reconnection, and turbulence. Recent observations from SDO and SOHO have provided insights into coronal stratification, showing that the corona's density decreases rapidly with height, consistent with the observed temperature gradient. SDO's AIA has revealed signs of magnetic reconnection and turbulence in the lower corona, contributing to plasma heating. Hinode’s spectral data show temperature and density variations across the corona, with observed coronal loops following a pattern where temperature increases with height in areas of strong magnetic activity. These observations support the idea that wave propagation and dissipation processes are responsible for maintaining the corona's stratified structure. High-resolution data from Hinode’s X-ray Telescope (XRT) and SDO suggest that magnetic fields play a crucial role in sustaining the observed stratification, channelling energy from reconnection events into localized high-temperature regions. The Global Oscillation Network Group (GONG) provides complementary ground-based data on solar magnetic activity, supporting these findings.
Magnetic Reconnection and Energy Transfer: Direct empirical evidence for magnetic reconnection in the corona comes from observations of solar flares and CMEs. These explosive events are associated with rapid energy release, indicating that magnetic fields play a key role in coronal heating. Instruments like Hinode and SDO have captured detailed images of magnetic structures in the corona, showing dynamic magnetic loops and fields that facilitate energy transfer. Hinode’s XRT has provided high-resolution X-ray images of reconnection jets, showing the release of energy that heats the plasma to millions of degrees. Observations from SDO reveal the rapid reconfiguration of magnetic field lines during solar flares, indicating magnetic reconnection. SOHO’s LASCO has observed CMEs linked to magnetic reconnection events in the lower corona and upper photosphere layers. Radio emissions from these events support the idea that reconnection accelerates particles, contributing to corona heating. These observations align with models suggesting that magnetic reconnection drives both localized heating and global energy transport in the corona. Recent numerical simulations based on observational data have provided further insights, indicating that reconnection rates vary depending on the magnetic field structure and turbulence, explaining why heating is uneven across the corona.
More Evidence and Observations: Empirical evidence supporting the role of nanoflares and magnetic reconnection in coronal heating has emerged from several space-based instruments and missions, including the Solar and Heliospheric Observatory (SOHO), TRACE (Transition Region and Coronal Explorer), and more recently, the Parker Solar Probe. These instruments have detected small-scale energy bursts that exhibit characteristics consistent with magnetic reconnection and nanoflare activity in the Sun's corona. One key piece of evidence comes from the detection of high-frequency oscillations and rapid changes in coronal heating that coincide with reconnection events. Instruments such as Extreme Ultraviolet Imaging Telescope (SOHO’s EIT) and TRACE have captured high-resolution images and spectroscopic data that reveal the dynamics of the corona in unprecedented detail. These observations show the presence of small-scale reconnection events, which are capable of releasing sufficient energy to heat the plasma to several million Kelvin, consistent with the temperature of the corona. As previously discussed, magnetic reconnection, wave heating, wave-particle interactions, and turbulence are the primary contenders for explaining the high temperatures observed in the corona. These mechanisms are capable of transporting energy from the Sun's photosphere to the corona, where it is dissipated as heat, overcoming the inefficiencies of traditional conductive heating models. The observational evidence supporting these mechanisms, including high-resolution images, spectroscopic data, and solar wind measurements, supports the conclusion that non-radiative processes (such as reconnection and wave heating) are primarily responsible for maintaining the elevated temperatures in the corona.
The Solar Cycle and Coronal Heating: The 11-year solar cycle of solar activity has long been known to influence the intensity and frequency of CMEs, solar flares, and sunspots, and recent studies have explored how this cycle affects the long-term heating of the corona. During periods of solar maximum, when solar activity peaks, coronal heating is significantly enhanced. The frequency of solar flares and the intensity of magnetic reconnection events in the corona increase during these periods, leading to greater heat dissipation and higher coronal temperatures. Parker Solar Probe observations have revealed a direct correlation between flare frequency and coronal temperature during solar maximum, suggesting that these events are a major driver of energy input into the corona.
Conversely, during the solar minimum, when solar activity is subdued, heating in the corona appears to be more diffuse, primarily driven by turbulent and wave-related heating mechanisms. Data from the SDO and Solar Orbitershow that, even though solar activity is lower, the corona maintains elevated temperatures through the persistence of small-scale heating processes like nanoflares and Alfvén waves. These non-flaring heating mechanisms remain crucial for sustaining coronal temperatures during quieter phases of the solar cycle.
Numerical Simulations and Wien's Law: Numerical simulations based on observational data have offered further insights into coronal heating mechanisms. For example, the application of Wien’s Law to the observed spectrum of the Sun has been used to study the coronal heating paradox. By comparing the observed spectral distributions from the photosphere, chromosphere, and corona, researchers have concluded that the energy output in the corona, especially in the UV and X-ray ranges, cannot be explained by traditional conductive heating models. Instruments like the Hinode EUV Imaging Spectrometer (EIS) have directly measured the spectral lines in the corona, supporting the idea that high-energy processes such as wave dissipation and magnetic reconnection are responsible for the observed temperature differences in the corona. High-resolution imaging from instruments like DKIST (Daniel K. Inouye Solar Telescope) and SDO has provided new insights into the role of small-scale magnetic structures, such as magnetic flux sheets and magnetic reconnection events, at the smallest scales of the corona. DKIST observations, in particular, have shown that small-scale magnetic structures (on the order of tens of kilometers) undergo rapid magnetic reconnection events. These events are responsible for both localized heating and the generation of coronal jets, suggesting that micro-scale events play a significant role in coronal heating. The dissipation of energy through these small-scale reconnection events has been found to be much larger than previously expected, providing new evidence that small-scale magnetic dynamics and wave-particle interactions contribute significantly to the heating of the corona.
Nanoflares and Small-Scale Events: High-resolution imaging from SDO has provided compelling evidence for nanoflares. These are small, localized bursts of energy that are hypothesized to be the primary mechanism for the continuous heating of the corona. The cumulative effect of numerous nanoflares and microflares could account for the observed high temperatures in the corona. Spectroscopic observations from IRIS and SDO show emissions consistent with the occurrence of nanoflares, particularly in regions of the corona with intense magnetic field activity, such as around active regions and sunspots. These regions exhibit frequent magnetic reconnection processes, supporting the theory that small-scale magnetic reconnection events are crucial for sustaining the high temperatures of the corona. Detections of nanoflares are not just theoretical, it has been confirmed as a real phenomenon. Observations show that these small-scale reconnection events lead to significant local heating, raising the temperature of the plasma to several million degrees Kelvin. This localized heating, resulting from magnetic reconnection events, contributes to the overall heating of the corona and may provide a solution to the coronal heating paradox by explaining the continuous energy input needed to maintain the high temperatures observed.
Scaling Laws and Observational Evidence: Empirical observations from Hinode, SDO, and SOHO offer direct evidence for many of the processes involved in coronal heating. By analyzing scaling laws derived from these observations, scientists have developed models to further support theoretical mechanisms of coronal heating. One such scaling law suggests that magnetic field strength and plasma density should be correlated with the observed coronal temperature. The empirical scaling law for the coronal temperature, with respect to magnetic field strength and plasma density, can be expressed as: T_corona = (B² * n)^α , where aα is a constant derived from observational data. B is the magnetic field strength and n is the plasma density. This scaling law indicates that regions with stronger magnetic fields and lower plasma density, such as active regions and coronal holes, should exhibit higher temperatures, which is consistent with observations. Coronal holes, which are areas of the Sun’s atmosphere with lower-density plasma and weaker magnetic fields, have been shown to maintain elevated temperatures, contrary to expectations based on their sparse magnetic activity. Recent observations from Solar Orbiter have revealed that the outflows from these coronal holes, which contribute to the solar wind, are thermally enhanced through local heating mechanisms. Studies using radio imaging techniques have shown that even though these regions lack intense flare activity, they still exhibit persistent low-level heating, driven by small-scale reconnection and wave-induced processes.
Solar Flares and Large-Scale Events: During solar flares, the release of magnetic energy leads to a sharp increase in the flux of non-thermal electrons, which can be directly observed through hard X-ray emissions captured by high-tech instruments like the Ramaty High-Energy Solar Spectroscopic Imager (RHESSI). These observations confirm that non-thermal electrons play a significant role in heating the plasma during flare events. SDO's AIA and RHESSI have captured detailed imaging and spectroscopy of flares, revealing how the release of magnetic energy accelerates particles and heats plasma to tens of millions of degrees within minutes. The relationship between solar flares, CMEs, and coronal heating is also evident in space weather data. Observations from space weather missions, such as NOAA’s DSCOVR and ACE, have measured the impact of CMEs on Earth's magnetosphere, showing how energy is transferred from the corona into interplanetary space. These events provide crucial opportunities to test theories of coronal heating in real-time, as they involve large-scale energy release through magnetic reconnection.
Spectroscopic Observations: Spectroscopic data from Hinode, SOHO, and SDO provide key insights into the high temperatures in the corona. For example, spectral lines from highly ionized elements such as Fe XII (iron) and Si VII (silicon) provide direct measurements of the ionization state of plasma at different altitudes in the corona. These measurements confirm the high temperatures required for the proposed heating mechanisms, particularly in regions with strong magnetic fields. Coronal seismology, which analyzes the oscillations in coronal loops, has further supported the idea that MHD waves contribute significantly to coronal heating. Wave energy dissipation plays a major role in transferring energy from the photosphere to the corona, and SDO’s AIA and Hinode’s EIS have revealed that coronal loops experience non-equilibrium heating during flare events and magnetic reconnection processes. Spectroscopic data from IRIS have also shown elevated electron densities and temperature gradients in coronal loops, providing direct evidence that localized heating within these structures contributes to the overall heating of the corona.
Solar Radiation and Radiative Processes: Observational data from the Hinode, SDO, and SOHO have provided substantial evidence that the corona is heated through radiation and non-radiative mechanisms. The high-resolution imaging provided by these missions shows dynamic, complex magnetic structures that indicate the role of magnetic fields in the coronal heating process. Furthermore, SOHO's ultraviolet and X-ray observations reveal significant emission from the corona, which is consistent with very high-energy processes occurring in the corona, rather than simple radiative processes. Data from the Hinode satellite’s X-ray telescope also support the idea that heating occurs in localized regions of the corona, where magnetic fields are intense and dynamic. Cronal seismology has provided evidence for the presence of waves which contribute to the heating processes. These waves can transport large amounts of energy from the photosphere and lower layers of the Sun’s atmosphere to the corona, where they dissipate, contributing to the observed temperature rise. By analyzing wave patterns observed by instruments such as Hinode and SDO, scientists have confirmed the presence of Alfvén waves and magnetoacoustic waves, which are thought to be important contributors to the heating of the corona. These waves are observed to propagate from the photosphere upwards into the corona, with their energy being transferred through wave-particle interactions and resonant absorption mechanisms.
Supergranulation and Transition Regions: The role of supergranulation in coronal heating has become increasingly important in recent research. Supergranules, which are large convection cells observed on the Sun’s surface, may influence the flow of energy from the photosphere to the corona, affecting the mechanisms responsible for coronal heating. Hinode and SDO have provided high-resolution images of supergranular motions, which reveal that these large convection cells could enhance the transport of energy to higher layers of the solar atmosphere, including the transition region and the corona. These motions may facilitate the generation of Alfvén waves and magnetoacoustic waves in the photosphere, which then propagate upward into the corona, carrying energy and contributing to the overall heating of the solar atmosphere. While the direct influence of supergranulation on the heating of the corona remains an active area of research, it is clear that the convective flows at the surface of the Sun play an important role in generating the waves and turbulence that drive coronal heating. The flow of energy from the photosphere to the corona through supergranular motions adds to the complexity of coronal heating mechanisms, contributing to the cumulative energy deposition that sustains the high temperatures observed in the corona.
The Role of the Transition Region in Coronal Heating: The transition region is the narrow layer between the Sun's chromosphere and corona. It has been found to play a crucial role in the process of energy transfer from the lower layers of the solar atmosphere to the corona. This region is characterized by rapid temperature changes, from about 10,000 K in the chromosphere to over a million degrees Kelvin in the corona. Understanding the dynamics of the transition region is essential for explaining how energy is transported across these layers, feeding into the heating of the corona. Recent observations from instruments like IRIS and SDO have revealed that the transition region undergoes significant heating events, which contribute to the overall heating of the corona. Detailed spectroscopic data from these instruments show that plasma in the transition region is rapidly heated over very small spatial scales. This rapid temperature increase is consistent with wave heating and the interaction of plasma waves in the chromosphere, where energy is transferred upward to the corona. The transition region's unique structure presents a significant challenge for solar physicists. Due to its narrow spatial scale and sharp temperature gradients, studying this region requires extremely high-resolution instruments. IRIS, for example, is capable of capturing emission lines from the chromosphere and transition region, providing valuable data on the energy transfer processes that occur in these critical layers of the solar atmosphere. The sharp gradient in temperature from the chromosphere to the corona suggests that the transition region is a key zone for energy dissipation and conversion, where energy from the photosphere is rapidly transferred into the corona.
Some Future Directions and Observational Challenges: Despite the significant advances in our understanding of coronal heating mechanisms, many aspects of the process remain uncertain. Key questions remain about the relative contributions of different heating mechanisms, such as nanoflares, wave heating, magnetic reconnection, and turbulence, and how these mechanisms interact on both small and large scales to maintain the high temperatures of the corona. Future missions and observations will be crucial for refining our models of coronal heating. The Parker Solar Probe, Solar Orbiter, and IRIS are already providing invaluable data, and upcoming instruments such as Daniel K. Inouye Solar Telescope (DKIST) will offer even higher-resolution imaging, allowing scientists to study the Sun's atmosphere with unprecedented detail. Parker Solar Probe, in particular, will provide a closer look at the Sun’s outer atmosphere, providing direct measurements of the solar wind, magnetic fields, and heating processes in the corona. One major challenge moving forward is integrating the various observational data with numerical simulations to create a comprehensive model that can explain the long-term behavior of the corona's temperature and the energy transport processes that sustain it. By combining data from space-based observatories, ground-based telescopes, and new solar missions, scientists are poised to uncover more detailed insights into the coronal heating paradox and its resolution.
Turbulence and Energy Dissipation: High-resolution data from Hinode and SDO have revealed small-scale turbulence within coronal loops, linked to magnetic reconnection events. These turbulent motions, observed as oscillations and plasma movements, provide direct evidence of turbulence in the corona. Regions of intense turbulence are often associated with high-energy flares and localized heating. Hinode’s EIS has shown signs of turbulence, with spectral line broadening as a signature. This broadening results from Doppler shifts caused by turbulent plasma motions, confirming that turbulence plays a significant role in energy dissipation in the corona. Numerical simulations based on these observations indicate that turbulence amplifies magnetic reconnection, increasing energy transfer from large-scale structures to smaller heated regions. Turbulence also contributes to the formation of small-scale current sheets where energy is dissipated and transferred to the plasma. These findings suggest turbulence is crucial in both short- and long-term coronal heating. SDO's coronal seismology data show oscillations likely driven by turbulence, contributing to energy dissipation. This evidence supports the idea that turbulence not only results from energy processes in the corona but also helps dissipate energy, fueling the heating of the solar atmosphere. Further data from Hinode’s SOT and SDO reveal turbulent features correlated with localized heating. Small-scale turbulence, including eddies and vortices in the corona and solar wind, contributes to energy dissipation and the heating process. IRIS data also show turbulent motions in the transition region between the chromosphere and corona, feeding energy into the corona. This highlights turbulence's role in the solar atmosphere's dynamic behavior and sustained coronal heating. Numerical simulations further suggest that small-scale turbulence can heat both electrons and ions, potentially explaining the high temperatures of the corona despite its low plasma density.
Wave Propagation and Energy Transport: Hinode and SDO provide key evidence of wave propagation through the corona. Hinode’s EIS detected both Alfvén and magnetoacoustic waves, supporting the theory that these waves are crucial for energy transport to the corona. Doppler shifts observed in spectral lines indicate wave motion and energy transfer. Non-thermal broadening of emission lines suggests waves energize the plasma through resonant interactions. SDO’s AIA captured high-resolution images of coronal loops, showing waves propagating along them, confirming their role in coronal heating. Studies using solar seismology techniques have confirmed that wave patterns in the corona match predictions from magnetohydrodynamic (MHD) models, proving waves can transport energy from lower layers to the corona. One significant observation from SDO is the detection of high-frequency oscillations in coronal loops, matching the frequencies of Alfvén waves. These waves likely dissipate energy via resonant absorption, transferring their energy to plasma particles as wave amplitudes increase. Additionally, SOHO detected propagating disturbances in the corona, confirming that waves cause local heating through non-linear processes like wave breaking and current sheet formation. IRIS observed wave motion in the transition region between the chromosphere and the corona, showing that waves carry energy to the corona. SOHO’s CDS also detected wave-like phenomena in the transition region, reinforcing the role of waves in energy transfer to the corona. Wave heating models are further supported by observations of coronal mass ejections (CMEs) from SOHO and STEREO, which show wave-like structures and energy transfer from lower layers to the corona and interplanetary space. Numerical simulations based on SDO observations demonstrate that wave propagation efficiently transports energy across the Sun’s atmospheric layers, offering a potential solution to the coronal heating paradox. Coronal seismology from Hinode and SDO shows a relationship between wave energy and temperature distribution in the corona, providing empirical evidence of the energy transport mechanisms responsible for coronal heating. Instruments on Parker Solar Probe, such as FIELDS and SWEAP, also support the idea of wave-particle interactions contributing to coronal heating, with wave dissipation leading to plasma heating. Spectroscopic measurements of the solar wind by IRIS show that waves accelerate electrons, which may further contribute to the high temperatures of the corona.
#academics#artistic#artwork#astronomy#beauty#corona#coronal#corona paradox#curiosity#creativity#core#discovery#discoveries#energies#forces#gravity#heating#innovation#key study#papers#physics#scientific#solar science#research#study#sun#sunlight#sunswater
1 note
·
View note
Text
Studie zeigt, dass die COVID-19-Injektion von Pfizer MEHR ALS 500-mal zulässige DNA-Kontamination aufweist https://behoerdenstress.de/studie-zeigt-dass-die-covid-19-injektion-von-pfizer-mehr-als-500-mal-zulaessige-dna-kontamination-aufweist/
0 notes
Text
Does Alternative Medicine Have a Future?
by Dr.Harald Wiesendanger– Klartext What the mainstream media is hiding The more effective alternative medicine is, the more popular it is and the more likely it is to threaten its continued existence. Because their success endangers the profits of an overpowering opponent who can destroy them. Their humble future lies in the health economics niche where word of mouth leads – under the radar of…
View On WordPress
#Alternative Medicine#Clinic#complementary medicine#Controlled Study#Corona#Corona crisis#Digital Services Act#Disinformation#Evidence#Fact Checker#Fake News#Foundation for ways out#Fourth Power#Future of Medicine#Harald Wiesendanger#Health Care#healthcare system#Hygiene Dictatorship#Integrative Medicine#pandemic#Private Doctors#RCT#Reporting Office#Science#social media#WHO#World Health Organization
0 notes
Text
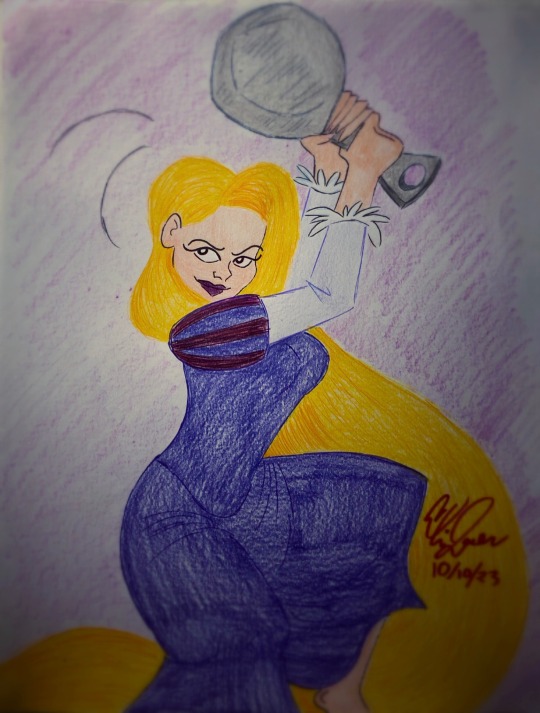
🌼🌸 Rapunzel is ready to defend herself, by any means necessary! 🎨🦎🐴
#Rapunzel#Tangled#Tangled Ever After#Tangled the Series#Rapunzel’s Tangled Adventure#Disney fanart#character study#fighting stance#frying pan#Kingdom of Corona#Snuggly Duckling#the Dark Kingdom#Eugene Fitzherbert#Pascal#Maximus#Mother Gothel#Queen Arianna#King Frederic#Cassandra#When Will My Life Begin#I See the Light#Mandy Moore#Glen Keane#late night drawing#Jin Kim#The Brothers Grimm#fairy tales#Disney Revival era
6 notes
·
View notes
Text
S.W || SILK & SHAPESHIFTERS
Sam Winchester x Thick!Fem!Reader
Content Warning reader being shorter than Sam, reader desc as having thick thighs, dean being annoying, swearing, reader wanting to fuck sam (but no sexual content in this fic)
Summary Fluff, (Sexual tension lowk) strangers to acquaintances to lovers(?) - You didn't work in the field. You did research. But when your good friend Bobby Singer asks you to help some friends of his by posing as a couple at a charity ball, you don't refuse.
W.C. 2.2k words
Ask anon: A: Hello hello! I'm back again; I'm going to change my request style a bit, how about a female reader with thick thighs? Make her with Sam please
Playlist: ♫ Bed Chem - Sabrina Carpenter, Shoot to Thrill - AC/DC
A.N. sam fic ! i am curvy so i was excited asf for this req !!! part 2 coming soon... ;) - claire xx
Being a Lore-keeper has its perks. It let you put your literary degree to some use, and it didn't usually get you into too much trouble. But, tonight it had. Bobby Singer was one of your favorite hunters; always treated you well, housed you in sticky situations, and sometimes had books or information you didn’t know about that he offered for you to borrow. Usually, he came to you for information. He didn’t hunt a lot, but it was still more than you hunted. But, when he and his hunter friends did go out looking for trouble, he often came to you for information. When he’d asked you to help out some of his family on a case that was real serious, you agreed begrudgingly. But only after Bobby told you your outfit, dinner, and drinks that night would be paid for by this said ‘family’ of his.
You’d already done a bit of research for him about this particular case and even you knew it was a tricky one. You’d spent the last week trying to piece all of the information together — running it through your countless trusted lore books, sites you favored, and even paper clippings and newspapers from the library. The place in question was only a few hours from Bobby’s in some fancy estate you’d mapped out by memory the last few days. You were guessing it was your least favorite of the supernatural creatures you’d perilously studied — shapeshifters. They made your skin crawl, and were tricky when they came in multiple. The family you suspected they were impersonating were the Bradys, a rich family that had lived in South Dakota for generations and hosted fundraiser parties each year for some organization their rich friends ran. Some of the members of the family had been photographed partaking in some suspicious activity, activity of which the police deemed fine of course; but you were smart enough to know this was your type of problem. A few of them had also seemingly gone missing, and either mysteriously turned up fine, or were still a missing persons case.
You decided to use Bobby’s as a place to get ready the second he offered, he lived closest anyways. You thought it was best to come over in the morning to talk more to him and whoever you were going on the case with, plan everything out. He’d been vague about who it was, though, and when you walked inside and saw Sam and Dean Winchester holding suits, a shopping bag, an EMF reader, and a pack of Coronas, you knew why.
You’d never given the boys information directly — Bobby had told you he relayed much information from you to them in tricky cases, but that the older one, Dean, was too prideful to ask you directly yet. Of course, like all male jackass hunters, he had eventually asked when he learned what they were dealing with and all the intricacies that you were extremely skillful in. The catch was that you hated field work, but also loved an excuse to get dressed up. You’d told Bobby your dress and shoe size, and sure enough, the taller one was holding a dark velvety blue dress over his arm.
“y/n, this is Sam, and this is Dean.” The shorter one, though he towered over you still, stuck out his hand, that jackass smirk on his face you knew he’d have.
“Heard a lot about you.” He spoke, the smirk still on his, admittedly handsome, face.
You didn’t like having your guard up. You hated it really, but being in your line of work and constantly dealing with men who dismissed your intelligence made you rightfully put up walls when you’d first meet them.
“Heard a lot about you too, but don’t think that means I’m your best friend now. I’m doing this for Bobby.”
The taller one smiled gingery, letting out a silent laugh watching Dean’s ego deflate. You turned to him, sticking out your hand. “Sam. Nice to meet you. And uh, thanks for coming out on this case, Bobby said field work isn't usually your thing so we really appreciate it.” You already preferred him. A lot, actually. He was tall, and looked way too fucking cute in that oversized brown hoodie he still had on despite being inside Bobby’s fairly warm home.
“We’re taking off at five so we’ll be right on time, okay?” Dean said to the two of you, rolling his eyes at how you hadn’t made any sassy comments at his brother.
“Sounds good. May I have my items, gentleman? I’m not doing this for free.” Sam smiled again, more noticeably this time, handing you the dress and shoes, and a purse, which you hadn't asked for but were not going to turn down.
“Thank you. So, the game plan is…?” “Game plan is you and I pose as a date and distract people while Dean sneaks around and corners a couple shifters, ganks ‘em, and then we get out before all hell breaks loose.”
You shrugged, looking at Sam, “Sounds good to me.” Of course he was cute and smart. God, you usually never let some guy get between you and case work, but he was making it hard. But if all you had to do was pose as a couple…
“Wait. If you needed me for acting I don’t know why you couldn’t call someone else.”
“We don’t know who's a shifter and who isn’t. That’s the problem. You know their tells, what makes them tick, how to trick them, the layout of the place. Bobby's got some ear pieces for all of us so you can help me from afar, don’t have to get your hands dirty.” Dean grunted, losing his dark leather jacket and grabbing a suit, heading to the bathroom to change.
“How thoughtful,” you mused, and he smiled annoyingly at you.
“We’ll talk more in the car, okay? Just get ready and we’ll be here if you have any more questions.” Sam said softly. HIs voice was deep and smooth, and it made you want to grab him by his collar and lock the two of you in Bobby’s guest room. Instead, you opted for pushing your thighs together and clearing your throat. “Sounds good. Thank you.” He smiled, going into the guest room with his own suit. You opted to lock yourself upstairs in the bathroom blasting music while you did your makeup in order to pump yourself up. You were a bit nervous, but you didn’t have to do dirty work, you got a nice new outfit, hopefully a nice drink, and a hot date? This was much better than how you thought your night was going to go — curled up on the couch, looking through way too many old files and books for another hunter, playing an old movie in the background and wishing you were elsewhere. You liked your job, you really did. But sometimes it drove you crazy how little you saw other people, people your own age especially. Tonight was like a gift sent from the angels. Well, ones that you hadn’t met; so far they had all been dicks.
“y/n, ya’ almost ready?” Dean called up, just as you finished styling your hair in a classy style.
“Coming!” You came down the stairs in a navy dress that hugged your curves pretty well, you thought. The dress was pretty low cut and the sides came up at a slit high up on your right leg. You honestly felt exposed, you usually didn't dress like this. Sam still had your heels, and when you came down, the first thing you saw was his eyes on your legs, stopping him from tying up his shoes.
You heard a low whistle behind you, and Dean’s gruff voice, “Damn, you clean up nice.” Bobby smacked him over the head with the book he was reading, going to the kitchen to get you an earpiece he had for each of you.
“You don’t look so bad yourself.” You smiled, walking over to Sam on the couch. He was still occupied with your dress, and his eyes were making your face burn up. Your brain couldn't decipher how he was feeling based on his face… you teetered between him wanting to rip off your dress and stay home with you all night, or that he thought you looked…not good. You knew you didn't have skinny model legs, but that wasn't you job. Your job was to decode, translate, find, and relay important information to hunters, stopping people from dying. That was you job, and you were good at it. There was so much more to you than your looks, and if someone couldn't see that then fuck them. But, with how stuffy the room was and how close Sam was to you, you wrapped your arms around your shoulders, sitting a bit far from Sam asking for your shoes despite the voices in your head telling you you were fine.
“Y-yeah, here. I’ll put them on.” Your mouth opened to speak, but it was dry and nothing came out. Sam leaned down from the couch, sitting on his knees right in front of you. You finally stretched out your left ankle, and he slid on one of the black stilettos they'd picked out for you. He set down your foot after buckling the straps, grabbing your right ankle and moving it forward gently, causing the fabric of your dress to fall between your thighs on one side, and on the very back of your hip on the other, your leg completely bare. Sam finished tying up your second shoe, his eyes flashing to your soft thigh before clearing his throat and offering a hand to help you stand up.
Once you were stable, you flattened down your dress. You looked up at Sam and pouted at how much taller he still was than you.
“Aren’t these like…almost 4 inches?” Sam looked down at you and chuckled deeply.
“Uh, yeah. They are. Disappointed you're still shorter than me?” You rolled your eyes and glimpsed over him — eyes trailing his fitted black suit, the fabric clinging to his chest and legs nicely.
“What are you, like part giant? 7 feet tall?” You said it as a joke, but you honestly thought he had to be close to that.
“Please,” Sam said, a bit close to your ear, leaning down to talk to you, “I’m…6’5. Just about.”
“Jesus…” you muttered, and realized you’d said it outloud. “Uh, we should get going soon, I think, it’s almost five, right?”
Sam nodded, squinting his eyes at your lower body so quickly you almost missed it. Dean put ear pieces in each of your hands and showed you how to use them. You followed the brothers out to their car and said bye to Bobby.
“Be careful, idjits. Keep in contact.”
“Will do.” You said, smiling to Bobby before getting in the back of the car, Sam opening and closing the door for you before sliding into the front seat a bit awkwardly from how long his legs were.
“Ready?” Dean uttered, putting his elbow on Sam’s seat.
“As ever.” You tucked a piece of hair behind your ear, seeing Dean’s vividly green eyes through the rear view mirror. Shoot to Thrill by AC/DC came on, and Sam mused on your quiet singing.
“You like AC/DC?” Sam asked, turning around a bit to talk to you more clearly.
“Yea, that, Metallica, some Guns N’ Roses, Rolling Stones....” Sam groaned, rolling his eyes, knowing Dean would have some stupid comment about that.
“Really?” Dean spoke up, turning down the music a bit.
“Yes. Don’t cream your pants.” Sam smiled at that. It wasn’t super often that women Dean hit on shut him down as unreservedly as you did.
“I know you got eyes for my idiot brother, but at least entertain me.”
“Okay. Who was the one that picked out the dress? And shoes? And purse?” You smirked at the back of Dean’s head.
“Sammy…” Dean mumbled begrudgingly.
“That's what I thought. Can you turn the music back up?” Dean’s eyes nearly bugged out of his head. He’d never had someone come in his car and tell him to do something like that, but he did so without saying anything.
Hours passed, and the Impala rolled to a stop in front of the biggest most obnoxious house you’d ever seen.
“Jesus. Showoff much?” Dean voiced from the front seat, unbuckling and stepping out. Sam followed, opening your door before you got the chance.
“So civilized.” You said poshly, seeing that adorable smile you liked seeing on Sam’s beautiful face.
“Alright. You two go inside first. Use the earpiece to tell me when you know where one is, tell me which way to go. We don’t know each other, got it? You got your fake, y/n?” said Dean.
“My what?” Sam handed you an ID with a photo of your face, but it didn’t say your name. “How many of these do you guys have? Actually, don’t answer that.” Sam held out his right arm and you slid yours under his. He began walking, taking large spread out steps. You saw his face flash, knowing he realized you were struggling to keep up; not just from your heels, but from his obnoxious height.
“Sorry,” he muttered, again, with that deep, smooth voice close to your ear. Fuck. This is gonna be a hard night.
#supernatural#supernatural masterlist#dean winchester#sam winchester#castiel#charlie bradbury#dean winchester x reader#sam winchester x reader#supernatural fanfiction#fanfiction#supernatural fluff#supernatural angst#supernatural smut#sam winchester angst#sam winchester x angel reader#supernatural x reader#sam winchester x reader angst#sam winchester x plus size reader#sam winchester x curvy reader#sam winchester x thick reader
516 notes
·
View notes
Text
Theoretical and Practical Solutions for the Coronal Heating Paradox
The coronal heating paradox is or was one of the most profound challenges in solar physics, rooted in the observation that the Sun’s corona is significantly hotter than its underlying photosphere. The photosphere, with a temperature around 5,500°C, contrasts sharply with the corona’s temperature, which can reach several million degrees. This discrepancy in temperature, despite the photosphere being the layer closest to the Sun’s inner layers, which also can be very hot, defied early models of solar energy transport. Recent advances in understanding solar winds, solar particle physics, magnetic field dynamics, relativistic particle acceleration, and wave-particle interactions have provided a comprehensive framework for explaining the heating mechanisms involved in the corona. This summary of the Sun’s Water Theory expands on some core mechanisms that collectively address the coronal heating paradox, with a focus on new insights from recent research, refined models, and theoretical approaches.
Detailed Analysis of the Key Factors Driving Coronal Heating
Acceleration of Solar Wind Particles: The solar wind, a continuous outflow of charged particles from the Sun, plays a critical role in coronal heating. These particles, which include electrons, protons, and heavier ions, are continuously accelerated away from the Sun by the magnetic field and pressure gradients within the corona. As these particles travel through the coronal and interplanetary space, their speeds increase, with some reaching near-relativistic velocities. The coronal heating paradox can be explained by the acceleration of these particles to extreme velocities near the speed of light. The relationship between the coronal temperature and the velocity of these particles is governed by the kinetic energy-temperature connection - higher temperatures increase the kinetic energy of particles, which accelerates them. As these high-energy particles travel along magnetic field lines, they gain further energy and speed. If they collide in the corona, their energy is released in the form of heat, which sustains the high temperatures.
Gravitational Effects and Plasma Dynamics: Though the Sun’s gravitational field becomes weaker at the outer layers of the solar atmosphere, it still plays a subtle role in the acceleration of solar wind particles. The gravitational potential energy of these particles is gradually converted into kinetic energy as they move outward. This effect, although small, adds to the overall energy budget of the solar wind. As particles are accelerated outward, their velocity increases, and their kinetic energy contributes to the heating of the plasma in the corona. The behavior of the plasma, including its motion and interactions with the magnetic field, also affects how energy is distributed across the corona. The dynamical behavior of plasma, driven by temperature gradients, magnetic forces, and gravitational effects, facilitates the creation of regions of concentrated energy, where particle collisions and reconnection events occur more frequently, enhancing the heating.
Magnetic Feedback Loops and Continuous Acceleration: Magnetic loops play a crucial role in sustaining the high temperatures of the corona. During solar flare events, the Sun's magnetic field can become highly structured, forming closed loops that trap energetic particles. These feedback loops result in continuous particle acceleration as the trapped particles spiral around the magnetic field lines, repeatedly gaining energy with each pass. The closed magnetic loop model demonstrates how magnetic reconnection events lead to feedback mechanisms that cause further particle acceleration and heating. The feedback mechanism is also linked to the presence of plasma waves within the corona, which further enhance particle motion. For example, Alfven waves propagate along the magnetic field lines and interact with the plasma, accelerating the particles and transferring energy to the corona. These waves facilitate the continuous supply of energy necessary to sustain the high temperatures in the corona, explaining the persistent heating despite the absence of a direct thermal connection with the Sun’s surface.
Magnetic Field Dynamics and Reconnection: One of the primary mechanisms for coronal heating is magnetic reconnection. Magnetic fields in the corona undergo frequent reorganization, especially during intense solar events such as solar flares and CMEs. In this process, opposing magnetic field lines from different regions of the Sun’s atmosphere reconnect, releasing vast amounts of energy that accelerates charged particles (mainly electrons and protons) to high velocities. These accelerated particles then contribute to the heating of the corona through collisions and the release of radiation. Magnetic reconnection is most effective when field lines form closed loops during flares, creating feedback loops that trap and accelerate particles repeatedly, sustaining high-energy environments. These reconnection events lead to an enhancement in the local magnetic field energy, which is converted into thermal and kinetic energy, directly contributing to the temperature increase of the corona. The solar flare model, which shows that the release of energy during magnetic reconnection can produce an X-ray and gamma-ray signature, is directly linked to the sudden and intense heating observed in the corona during such events.
Relativistic Particle Acceleration and Mass-Energy Relations: One of the key breakthroughs in understanding the heating of the corona is the role of relativistic speeds in the acceleration of solar wind particles. As particles are accelerated to relativistic velocities, their mass and energy increase according to the relativistic energy equation. This relationship, expressed in terms of the Lorentz factor (γ), significantly alters the energy dynamics in the corona. At near-light speeds, the kinetic energy of particles increases dramatically, and the potential for heat release during particle collisions also increases. The accelerated electrons and protons that reach relativistic speeds are responsible for much of the energetic radiation observed during solar flare events, such as X-rays and gamma-rays. These high-energy particles interact with the solar atmosphere, releasing energy that contributes to coronal heating. The increase in mass of relativistic particles is another critical factor: the higher the speed, the greater the mass, and thus the greater the energy released in collisions. This is a direct and logical explanation for the high temperatures observed in the corona, as relativistic particles can transfer substantial amounts of energy during these interactions.
Solar Activity Cycles and Temperature Variations: The intensity of coronal heating varies with the solar cycle, with periods of solar maximum corresponding to increased activity in the form of more frequent solar flares and CMEs. These events lead to higher temperatures in the corona, as the release of energy from magnetic reconnection events and particle acceleration becomes more intense. During solar minimum, when solar activity is low, coronal heating decreases, and the temperature of the corona drops. This variability underscores the importance of solar activity in regulating the heating process.
Thermal vs. Non-Thermal Contributions: Both thermal and non-thermal processes contribute to the heating of the corona. While traditional heating models focused on thermal conduction and radiation from the photosphere, recent research highlights the role of non-thermal processes, particularly the acceleration of high-energy particles during solar flares. These particles, once accelerated, can interact with the corona and release energy in the form of X-rays and gamma-rays, which significantly increase the local temperature. This dual contribution from thermal and non-thermal sources is essential to understanding the full energy budget of the corona.
Wave-Particle Interactions: The corona’s heating is further enhanced by wave-particle interactions. Waves, such as Alfven waves and slow magnetosonic waves, propagate through the solar atmosphere, transferring energy to the plasma. These waves interact with charged particles, providing them with additional velocity and kinetic energy. The process of wave-particle resonance accelerates the particles, particularly electrons, to high speeds. These interactions are most efficient in regions with strong magnetic fields, where waves are most energetic. As these waves move through the plasma, they generate turbulent heating, which is a key contributor to the high temperatures observed in the corona. The efficiency of wave-particle interactions is influenced by both the intensity of the waves and the density of the plasma. During active solar periods, when wave activity is high, the corona experiences more intense heating, reinforcing the link between solar activity and coronal temperature.
The Photosphere and the Temperature Discrepancy Between the Sun's Corona
The Sun’s photosphere is significantly cooler than its outer atmosphere, the corona, which reaches temperatures of several million degrees despite being farther from the Sun’s core. This temperature gradient has long puzzled solar physicists, and while the high temperature of the corona has been partially explained by magnetic reconnection and wave-particle interactions, the cooler photosphere presents a more subtle and multifaceted issue. The key to understanding this discrepancy lies in the energy transport mechanisms, physical conditions, and thermodynamic processes that govern both the photosphere and corona.
1. Convective Energy Transport in the Photosphere
The photosphere maintains a temperature of approximately 5,500°C (or 5.8 x 10³ K) due to its position in the solar interior's convective zone. The Sun’s energy is produced by nuclear fusion in the core, but by the time this energy reaches the photosphere, it has already undergone several stages of transport.
Convective Heat Transfer: Below the photosphere, the Sun's energy is transferred outward through convective currents. Hot plasma rises toward the photosphere, losing heat at the surface, and then cools and sinks back into the interior. This convective process is highly efficient at transporting heat, preventing the photosphere from reaching temperatures similar to those of the corona.
Empirical Evidence: Observations of the photosphere from instruments like SOHO and SDO confirm that the photosphere remains in a dynamic equilibrium, with convective cells observable on the solar surface (granulation patterns). These patterns indicate efficient heat transport and energy dissipation at the photospheric level, keeping it cool relative to the corona.
Opacity and Radiation: The photosphere also acts as a boundary where the Sun’s interior becomes optically transparent. Above this layer, energy is radiated outward through radiation diffusion, which further cools the surface. The transition from opaque to transparent occurs just below the photosphere, where energy is transported by radiative diffusion, a process far less efficient than convection.
2 . Energy Loss and Radiative Cooling
The temperature of the photosphere is also dictated by the balance between energy absorption from below and the energy radiated away into space. At this layer, energy transfer occurs via radiative cooling, which happens through the emission of photons as the hot plasma at lower levels moves toward the photosphere. This radiative process cools the plasma down significantly as it reaches the photosphere, preventing it from becoming as hot as the overlying corona.
Radiative Cooling: As the energy moves upward from the Sun's core, it loses energy due to the constant emission of photons at different wavelengths. By the time this energy reaches the photosphere, much of it has been diffused and cooled. Consequently, the photosphere’s temperature is much lower than that of the corona, which is dynamically heated by other processes beyond this level.
Thermal Balance: The photosphere's effective temperature is determined by the balance of energy received from the interior and energy radiated away into space. The effective temperature of 5,500°C is the result of this balance, with infrared radiation being the dominant cooling mechanism in the photosphere.
3. Magnetic Field and Lack of Heat Input in the Photosphere
The magnetic field in the photosphere plays a minimal role in heating compared to the corona. While the corona is strongly influenced by magnetic reconnection and wave-particle interactions, these processes are far less active in the photosphere regions. The photosphere is more of a thermal equilibrium layer where magnetic fields, though present, do not undergo significant dynamic processes like those in the corona.
Empirical Observations: Studies of sunspot activity and solar magnetic field configurations confirm that while the photosphere experiences solar activity, these events are not sufficient to explain the extreme temperatures observed in the corona. The solar magnetic flux in the photosphere is not strong enough to initiate the same heating mechanisms responsible for the corona's high temperatures.
Weak Magnetic Activity: The photosphere’s magnetic fields are relatively weak compared to the intense and highly dynamic fields present in the corona. While magnetic fields in the photosphere can lead to the formation of sunspots and active regions, they do not exhibit the same magnetic reconnection events or wave heating that occur in the corona. This lack of active magnetic processes means that the photosphere is less susceptible to the energy inflows that heat the corona.
4. Thermodynamic Differences Between the Photosphere and Corona
The thermodynamic properties of the photosphere and the corona are distinct, not only in temperature but also in how energy is transferred and dissipated. The corona is heated by non-thermal processes such as magnetic reconnection and wave-particle interactions, while the photosphere relies on thermal conduction and radiative diffusion.
Heat Loss in the Photosphere: The photosphere's temperature is regulated by thermal equilibrium; it cannot accumulate energy from below because the convective and radiative processes are highly efficient at transferring heat outward and cooling the plasma. In contrast, the corona, though farther from the Sun’s core, receives energy through external mechanisms like reconnection and wave heating, which are absent in the photosphere.
Energy Dissipation: The photosphere loses energy efficiently through radiation, with no external heating mechanisms like those that dominate in the corona. Thus, its temperature remains relatively cool compared to the corona, despite being closer to the Sun’s core. The distinction between the Sun's photosphere and corona is crucial to resolving the coronal heating paradox. To further elaborate on the mechanisms behind the photosphere’s lower temperature, we will dive deeper into the energy dynamics, magnetic field behavior, and empirical data that collectively contribute to this striking temperature disparity.
5. Solar Interior and Energy Transport to the Photosphere
In the interior of the Sun, nuclear fusion occurs at the core, releasing vast amounts of energy in the form of gamma-ray photons. These photons undergo a slow, multi-stage process of energy transport through the radiative zone before reaching the convective zone, where energy is transported outward by convection. By the time this energy reaches the photosphere, it has undergone substantial cooling.
Evidence from Sunspot Studies: Sunspots, which are areas of strong magnetic fields in the photosphere, are cooler than their surroundings. This is a clear indication that the convective energy transport is effective in regulating the photosphere’s temperature, even in the presence of concentrated magnetic activity.
Plasma Convection: The photosphere lies at the upper boundary of the Sun’s convective zone, where convective cells (granules) move hot plasma upward from deeper layers. As plasma rises toward the photosphere, it cools radiatively and loses energy. When the cooler plasma reaches the photosphere, it radiates that energy into space, and then sinks back down. This convective transport is highly efficient at maintaining a relatively low temperature for the photosphere, preventing it from reaching the extreme temperatures found in the corona.
Radiative Zone to Convective Zone Transition: The transition from the radiative zone (where energy is transported by photon diffusion) to the convective zone (where energy is transported by the bulk motion of plasma) marks a critical point in the solar energy transport system. As energy moves outward, the temperature decreases and the convective efficiency increases. This means that, by the time the energy reaches the photosphere, its ability to raise the temperature of the Sun’s outer layers diminishes significantly.
6. Absence of Magnetic Reconnection in the Photosphere
The magnetic field in the photosphere plays a significant role in the Sun’s overall activity but does not contribute directly to the heating of the photosphere itself. While the corona is subjected to magnetic reconnection, which accelerates particles and generates heat through wave-particle interactions, the photosphere does not exhibit the same dynamism.
Magnetic Flux in the Photosphere: The photosphere contains large-scale magnetic structures such as sunspots and active regions, but these fields are relatively static compared to the complex, rapidly evolving magnetic field structures found in the corona. In the photosphere, the magnetic field primarily acts to organize plasma into granules and supergranules, structures that do not directly contribute to heating through magnetic reconnection.
Magnetic Reconnection in the Corona: In contrast, in the corona, magnetic fields undergo frequent reconnection events. These events release substantial amounts of energy, particularly in the form of electromagnetic radiation, kinetic energy, and high-energy particle acceleration. The absence of such reconnection in the photosphere ensures that this layer remains cooler despite its proximity to the Sun's core.
Solar Observations: Observations from missions such as SOHO and SDO reveal the dynamic nature of the corona’s magnetic field, which constantly reconnects and reconfigures, leading to intense heating. These phenomena are far less prominent in the photosphere, where the magnetic fields are largely responsible for structure but do not contribute to significant energy dissipation.
7. Thermal Radiation and Efficient Energy Loss
The photosphere is a boundary layer in the Sun’s atmosphere where energy loss via radiation is optimized. The solar spectrum emitted from the photosphere consists primarily of visible light and infrared radiation, which is emitted as the photosphere's cooler plasma radiates energy away. This is a direct result of the Planck distribution and the Stefan-Boltzmann law, which govern blackbody radiation.
Empirical Data: Observational data, including spectral measurements from space-based telescopes, shows that the photosphere’s radiation is consistent with blackbody radiation laws at a temperature of about 5,500 K. The intensity and spectrum of the radiation from the photosphere confirm the dominant role of radiation in energy dissipation at this layer.
Energy Transport and Efficiency: The photosphere’s ability to radiate energy efficiently is crucial to maintaining its lower temperature. As energy reaches the photosphere, it is rapidly radiated away due to the relatively low opacity of the plasma at this level. The high opacity of the solar interior prevents this energy from escaping earlier in the solar layers, but once it reaches the photosphere, it is allowed to radiate efficiently into space, maintaining the cooler surface temperature.
Radiation Cooling: The temperature of the photosphere is in equilibrium with the rate of energy radiated away into space. The photosphere acts as a radiative boundary, ensuring that the energy produced deep inside the Sun is efficiently emitted at its surface. This cooling mechanism prevents the photosphere from reaching the temperatures observed in the corona, where non-thermal heating mechanisms dominate.
8. Photosphere’s Role in Solar Activity and Stability
While the photosphere remains cool compared to the corona, it is still deeply interconnected with the Sun’s overall activity. The photosphere is the site of sunspot formation, which are cooler areas of concentrated magnetic activity. These sunspots form in regions where the Sun’s magnetic field is particularly strong and act as markers of solar activity cycles.
Sunspot Temperature Differences: The temperature of sunspots is typically about 3,000-4,000°C, considerably cooler than the surrounding photospheric plasma. This temperature difference is a direct consequence of the magnetic fields in the photosphere inhibiting the normal convective energy transport, creating local thermal inversions.
Solar Cycle and Activity: The photosphere is also where the solar cycle, the 11-year period of solar magnetic activity, manifests as periodic changes in the number of sunspots and active regions. The solar cycle influences the photosphere's overall appearance but does not significantly affect its temperature, as the temperature is primarily controlled by the convective heat transport and radiative cooling processes mentioned above.
Integrating the Photosphere’s Role in the Resolution of the Paradox
The cooler photosphere is the result of complex but well-understood processes that regulate energy transport and dissipation. Convective heat transport ensures that energy from the Sun’s interior is efficiently carried to the photosphere, where it is radiated away. The lack of dynamic magnetic field behavior compared to the corona means there is no external source of non-thermal heating to increase the temperature of the photosphere. Additionally, the efficiency of radiative cooling at the photospheric level ensures that the surface temperature remains in equilibrium with the energy that is radiated away into space. Empirical and observational evidence from solar missions, combined with theoretical models of heat transport and magnetic field dynamics, consistently support the conclusion that the photosphere’s cooler temperature is primarily controlled by convective cooling, radiative energy loss, and the absence of active magnetic heating mechanisms that dominate the corona. These differences in energy transport mechanisms, combined with the physical conditions and thermodynamic processes at play, explain why the photosphere remains significantly cooler than the corona. The integration of the Suns Water Study and advanced research papers for solar science, highlighting the crucial roles and key processes that finally solved the scientific mystery and physical problem - including many empirical evidences and very logical solutions.
Comprehensive and Short Summary
The solution to the coronal heating paradox lies in a multi-faceted approach that integrates magnetic feedback loops, magnetic reconnection, relativistic particle acceleration and wave-particle interactions within the Sun’s dynamic atmosphere. These processes, driven by solar activity such as solar flares and CMEs, release vast amounts of energy, heating the corona to millions (1~2m+) of degrees. The interplay between magnetic field dynamics and plasma motion, combined with the acceleration of particles to relativistic speeds, ensures that the corona remains hot, despite being far from the Sun’s core. Theoretical physics, mathematical models, observational and empirical evidence of research in solar science can confirm the mechanisms that drive coronal heating. Numerous observations from solar missions have shown that these processes are interdependent and play critical roles in sustaining the high temperatures of the Sun's corona even in times of low solar activity. This understanding not only resolves the apparent paradox of the cooler photosphere and hotter corona but also underscores the dynamic nature of solar energy transport across different layers of the Sun's atmosphere, integrating both thermal and non-thermal processes to explain the observed temperature variations. Through the integration of these phenomena, we now have a comprehensive understanding of how the corona achieves and maintains its temperature, resolving the apparent contradiction with the cooler photosphere beneath it. The author of the Sun’s Water Theory and study solved the corona paradox on many ways and with a comprehensive research chapter around solar science and physical processes in releation to solar physics. Many scientific evidences are explained on some of the over 50 pages concentrated and extended research. More details and backgrounds are in the latest preprint versions of November and will be published also in the next previews and pre-publications in December.
#academic#artistic#breakthrough#coronal#corona paradox#curiosity#discovery#educational#paper#scientific#science#solar#study#sun#sunswater#theory
1 note
·
View note
Text

perspective study that i decided to draw as corona bc the pose reminded me of her and then i struggled and i struggled and i struggled and i str
#i hate doin perspective like this bc u be getting like dysmorphia but for ur art like#im pretty sure this is jank but i just can't be bothered to fix it anymore so HERE#my art#procreate#the locked tomb#tlt#coronabeth tridentarius#nona the ninth#ntn#i find drawing her face so damn hard i hate drawing hot people
850 notes
·
View notes
Text
What if we're just a little off the mark, when it comes to Ianthe's goals? It does seem like she's trying to become god. Studying resurrection theory, energy transfers, trying to replicate John's stasis trick on those apples. But Ianthe has always either shied from or been denied the spotlight. And the path she's on starts with her specialising in Resurrection theory.
What was the Resurrection, other than the obvious? It was the first recorded act in history, the beginning of necromancy. Why would a girl playing power double for her non-adept sister want to learn the secrets of the Resurrection? Well, there are plenty of reasons, but one that comes to mind is to learn how to make someone a necromancer who isn't. The first necromancer had to have gained the power somehow, right? Can you control who is and isn't a necromancer, if you're willing to pay the price? And then there was the conversation between Coronabeth and Judith, where they as much as said Corona could be king, but for the lack of job openings.
Whether she knows it yet or not, I suspect Ianthe's long-term plans are bending toward making Coronabeth god. All because she didn't want to keep doing her sister's homework.
#the locked tomb#ianthe tridentarius#coronabeth tridentarius#htn spoilers#harrow the ninth#as yet unsent#ayu spoilers
2K notes
·
View notes