#Scintillation Detector.
Explore tagged Tumblr posts
Photo

Radiation Area Monitors
The Radiation Alert® line of radiation area monitors protects your personnel and your facility. Select the right model to detect across a wide range of radiation levels. The base controller unit includes a Touch Screen LCD, latching, siren light and strobe. Internal or external radiation probes are available for most models, with Geiger Muller or Sodium Iodide Probe options.
Here are the Different Version of Radiation Area Monitors
AM-1x1 Radiation Area Monitor
Radiation Alert® Area Monitor is one of the most user friendly and it comes with either an internal or external Sodium Iodide Scintillation.
AM-2x2 Radiation Area Monitor
AM-2X2 comes with an external Sodium Iodide Scintillation Detector. Cesium Iodide probes available. Rugged, wall mount enclosure options are available for high traffic area.
AM-7128 Radiation Area Monitor
It comes with either an internal or external detector and free software for monitoring every Radiation Alert® Area Monitor on your network.
AM-71313 Radiation Area Monitor
AM-71313 comes with either an internal or external detector and free software for monitoring every Radiation Alert® Area Monitor on your network.
AM-7149 Radiation Area Monitor
It comes with either an internal or external detector and free software for monitoring every Radiation Alert® Area Monitor on your network.
0 notes
Text
Conceptual Design for a Neutrino Power Transmission System
Overview
Neutrinos could potentially be used to send electricity over long distances without the need for high-voltage direct current (HVDC) lines. Neutrinos have the unique property of being able to pass through matter without interacting with it, which makes them ideal for transmitting energy over long distances without significant energy loss. This property allows neutrinos to be used as a medium for energy transmission, potentially replacing HVDC lines in certain applications.
So the goal is to create a neutrino-based power transmission system capable of sending and receiving a beam of neutrinos that carry a few MW of power across a short distance. This setup will include a neutrino beam generator (transmitter), a travel medium, and a neutrino detector (receiver) that can convert the neutrinos' kinetic energy into electrical power.
1. Neutrino Beam Generator (Transmitter)
Particle Accelerator: At the heart of the neutrino beam generator will be a particle accelerator. This accelerator will increase the energy of protons before colliding them with a target to produce pions and kaons, which then decay into neutrinos. A compact linear accelerator or a small synchrotron could be used for this purpose.
Target Material: The protons accelerated by the particle accelerator will strike a dense material target (like tungsten or graphite) to create a shower of pions and kaons.
Decay Tunnel: After production, these particles will travel through a decay tunnel where they decay into neutrinos. This tunnel needs to be under vacuum or filled with inert gas to minimize interactions before decay.
Focusing Horns: Magnetic horns will be used to focus the charged pions and kaons before they decay, enhancing the neutrino beam's intensity and directionality.
Energy and Beam Intensity: To achieve a few MW of power, the system will need to operate at several gigaelectronvolts (GeV) with a proton beam current of a few tens of milliamperes.
2. Travel Medium
Direct Line of Sight: Neutrinos can travel through the Earth with negligible absorption or scattering, but for initial tests, a direct line of sight through air or vacuum could be used to simplify detection.
Distance: The initial setup could span a distance from a few hundred meters to a few kilometers, allowing for measurable neutrino interactions without requiring excessively large infrastructure.
3. Neutrino Detector (Receiver)
Detector Medium: A large volume of water or liquid scintillator will be used as the detecting medium. Neutrinos interacting with the medium produce a charged particle that can then be detected via Cherenkov radiation or scintillation light.
Photodetectors: Photomultiplier tubes (PMTs) or Silicon Photomultipliers (SiPMs) will be arranged around the detector medium to capture the light signals generated by neutrino interactions.
Energy Conversion: The kinetic energy of particles produced in neutrino interactions will be converted into heat. This heat can then be used in a traditional heat-to-electricity conversion system (like a steam turbine or thermoelectric generators).
Shielding and Background Reduction: To improve the signal-to-noise ratio, the detector will be shielded with lead or water to reduce background radiation. A veto system may also be employed to distinguish neutrino events from other particle interactions.
4. Control and Data Acquisition
Synchronization: Precise timing and synchronization between the accelerator and the detector will be crucial to identify and correlate neutrino events.
Data Acquisition System: A high-speed data acquisition system will collect data from the photodetectors, processing and recording the timing and energy of detected events.
Hypothetical Power Calculation
To estimate the power that could be transmitted:
Neutrino Flux: Let the number of neutrinos per second be ( N_\nu ), and each neutrino carries an average energy ( E_\nu ).
Neutrino Interaction Rate: Only a tiny fraction (( \sigma )) of neutrinos will interact with the detector material. For a detector with ( N_d ) target nuclei, the interaction rate ( R ) is ( R = N_\nu \sigma N_d ).
Power Conversion: If each interaction deposits energy ( E_d ) into the detector, the power ( P ) is ( P = R \times E_d ).
For a beam of ( 10^{15} ) neutrinos per second (a feasible rate for a small accelerator) each with ( E_\nu = 1 ) GeV, and assuming an interaction cross-section ( \sigma \approx 10^{-38} ) cm(^2), a detector with ( N_d = 10^{30} ) (corresponding to about 10 kilotons of water), and ( E_d = E_\nu ) (for simplicity in this hypothetical scenario), the power is:
[ P = 10
^{15} \times 10^{-38} \times 10^{30} \times 1 \text{ GeV} ]
[ P = 10^{7} \times 1 \text{ GeV} ]
Converting GeV to joules (1 GeV ≈ (1.6 \times 10^{-10}) J):
[ P = 10^{7} \times 1.6 \times 10^{-10} \text{ J/s} ]
[ P = 1.6 \text{ MW} ]
Thus, under these very optimistic and idealized conditions, the setup could theoretically transmit about 1.6 MW of power. However, this is an idealized maximum, and actual performance would likely be significantly lower due to various inefficiencies and losses.
Detailed Steps to Implement the Conceptual Design
Step 1: Building the Neutrino Beam Generator
Accelerator Design:
Choose a compact linear accelerator or a small synchrotron capable of accelerating protons to the required energy (several GeV).
Design the beamline with the necessary magnetic optics to focus and direct the proton beam.
Target Station:
Construct a target station with a high-density tungsten or graphite target to maximize pion and kaon production.
Implement a cooling system to manage the heat generated by the high-intensity proton beam.
Decay Tunnel:
Design and construct a decay tunnel, optimizing its length to maximize the decay of pions and kaons into neutrinos.
Include magnetic focusing horns to shape and direct the emerging neutrino beam.
Safety and Controls:
Develop a control system to synchronize the operation of the accelerator and monitor the beam's properties.
Implement safety systems to manage radiation and operational risks.
Step 2: Setting Up the Neutrino Detector
Detector Medium:
Select a large volume of water or liquid scintillator. For a few MW of transmitted power, consider a detector size of around 10 kilotons, similar to large neutrino detectors in current experiments.
Place the detector underground or in a well-shielded facility to reduce cosmic ray backgrounds.
Photodetectors:
Install thousands of photomultiplier tubes (PMTs) or Silicon Photomultipliers (SiPMs) around the detector to capture light from neutrino interactions.
Optimize the arrangement of these sensors to maximize coverage and detection efficiency.
Energy Conversion System:
Design a system to convert the kinetic energy from particle reactions into heat.
Couple this heat to a heat exchanger and use it to drive a turbine or other electricity-generating device.
Data Acquisition and Processing:
Implement a high-speed data acquisition system to record signals from the photodetectors.
Develop software to analyze the timing and energy of events, distinguishing neutrino interactions from background noise.
Step 3: Integration and Testing
Integration:
Carefully align the neutrino beam generator with the detector over the chosen distance.
Test the proton beam operation, target interaction, and neutrino production phases individually before full operation.
Calibration:
Use calibration sources and possibly a low-intensity neutrino source to calibrate the detector.
Adjust the photodetector and data acquisition settings to optimize signal detection and reduce noise.
Full System Test:
Begin with low-intensity beams to ensure the system's stability and operational safety.
Gradually increase the beam intensity, monitoring the detector's response and the power output.
Operational Refinement:
Refine the beam focusing and detector sensitivity based on initial tests.
Implement iterative improvements to increase the system's efficiency and power output.
Challenges and Feasibility
While the theoretical framework suggests that a few MW of power could be transmitted via neutrinos, several significant challenges would need to be addressed to make such a system feasible:
Interaction Rates: The extremely low interaction rate of neutrinos means that even with a high-intensity beam and a large detector, only a tiny fraction of the neutrinos will be detected and contribute to power generation.
Technological Limits: The current state of particle accelerator and neutrino detection technology would make it difficult to achieve the necessary beam intensity and detection efficiency required for MW-level power transmission.
Cost and Infrastructure: The cost of building and operating such a system would be enormous, likely many orders of magnitude greater than existing power transmission systems.
Efficiency: Converting the kinetic energy of particles produced in neutrino interactions to electrical energy with high efficiency is a significant technical challenge.
Scalability: Scaling this setup to practical applications would require even more significant advancements in technology and reductions
in cost.
Detailed Analysis of Efficiency and Cost
Even in an ideal scenario where technological barriers are overcome, the efficiency of converting neutrino interactions into usable power is a critical factor. Here’s a deeper look into the efficiency and cost aspects:
Efficiency Analysis
Neutrino Detection Efficiency: Current neutrino detectors have very low efficiency due to the small cross-section of neutrino interactions. To improve this, advanced materials or innovative detection techniques would be required. For instance, using superfluid helium or advanced photodetectors could potentially increase interaction rates and energy conversion efficiency.
Energy Conversion Efficiency: The process of converting the kinetic energy from particle reactions into usable electrical energy currently has many stages of loss. Thermal systems, like steam turbines, typically have efficiencies of 30-40%. To enhance this, direct energy conversion methods, such as thermoelectric generators or direct kinetic-to-electric conversion, need development but are still far from achieving high efficiency at the scale required.
Overall System Efficiency: Combining the neutrino interaction efficiency and the energy conversion efficiency, the overall system efficiency could be extremely low. For neutrino power transmission to be comparable to current technologies, these efficiencies need to be boosted by several orders of magnitude.
Cost Considerations
Capital Costs: The initial costs include building the particle accelerator, target station, decay tunnel, focusing system, and the neutrino detector. Each of these components is expensive, with costs potentially running into billions of dollars for a setup that could aim to transmit a few MW of power.
Operational Costs: The operational costs include the energy to run the accelerator and the maintenance of the entire system. Given the high-energy particles involved and the precision technology required, these costs would be significantly higher than those for traditional power transmission methods.
Cost-Effectiveness: To determine the cost-effectiveness, compare the total cost per unit of power transmitted with that of HVDC systems. Currently, HVDC transmission costs are about $1-2 million per mile for the infrastructure, plus additional costs for power losses over distance. In contrast, a neutrino-based system would have negligible losses over distance, but the infrastructure costs would dwarf any current system.
Potential Improvements and Research Directions
To move from a theoretical concept to a more practical proposition, several areas of research and development could be pursued:
Advanced Materials: Research into new materials with higher sensitivity to neutrino interactions could improve detection rates. Nanomaterials or quantum dots might offer new pathways to detect and harness the energy from neutrino interactions more efficiently.
Accelerator Technology: Developing more compact and efficient accelerators would reduce the initial and operational costs of generating high-intensity neutrino beams. Using new acceleration techniques, such as plasma wakefield acceleration, could significantly decrease the size and cost of accelerators.
Detector Technology: Improvements in photodetector efficiency and the development of new scintillating materials could enhance the signal-to-noise ratio in neutrino detectors. High-temperature superconductors could also be used to improve the efficiency of magnetic horns and focusing devices.
Energy Conversion Methods: Exploring direct conversion methods, where the kinetic energy of particles from neutrino interactions is directly converted into electricity, could bypass the inefficiencies of thermal conversion systems. Research into piezoelectric materials or other direct conversion technologies could be key.
Conceptual Experiment to Demonstrate Viability
To demonstrate the viability of neutrino power transmission, even at a very small scale, a conceptual experiment could be set up as follows:
Experimental Setup
Small-Scale Accelerator: Use a small-scale proton accelerator to generate a neutrino beam. For experimental purposes, this could be a linear accelerator used in many research labs, capable of accelerating protons to a few hundred MeV.
Miniature Target and Decay Tunnel: Design a compact target and a short decay tunnel to produce and focus neutrinos. This setup will test the beam production and initial focusing systems.
Small Detector: Construct a small-scale neutrino detector, possibly using a few tons of liquid scintillator or water, equipped with sensitive photodetectors. This detector will test the feasibility of detecting focused neutrino beams at short distances.
Measurement and Analysis: Measure the rate of neutrino interactions and the energy deposited in the detector. Compare this to the expected values based on the beam properties and detector design.
Steps to Conduct the Experiment
Calibrate the Accelerator and Beamline: Ensure the proton beam is correctly tuned and the target is accurately positioned to maximize pion and kaon production.
Operate the Decay Tunnel and Focusing System: Run tests to optimize the magnetic focusing horns and maximize the neutrino beam coherence.
Run the Detector: Collect data from the neutrino interactions, focusing on capturing the rare events and distinguishing them from background noise.
Data Analysis: Analyze the collected data to determine the neutrino flux and interaction rate, and compare these to
theoretical predictions to validate the setup.
Optimization: Based on initial results, adjust the beam energy, focusing systems, and detector configurations to improve interaction rates and signal clarity.
Example Calculation for a Proof-of-Concept Experiment
To put the above experimental setup into a more quantitative framework, here's a simplified example calculation:
Assumptions and Parameters
Proton Beam Energy: 500 MeV (which is within the capability of many smaller particle accelerators).
Number of Protons per Second ((N_p)): (1 \times 10^{13}) protons/second (a relatively low intensity to ensure safe operations for a proof-of-concept).
Target Efficiency: Assume 20% of the protons produce pions or kaons that decay into neutrinos.
Neutrino Energy ((E_\nu)): Approximately 30% of the pion or kaon energy, so around 150 MeV per neutrino.
Distance to Detector ((D)): 100 meters (to stay within a compact experimental facility).
Detector Mass: 10 tons of water (equivalent to (10^4) kg, or about (6 \times 10^{31}) protons assuming 2 protons per water molecule).
Neutrino Interaction Cross-Section ((\sigma)): Approximately (10^{-38} , \text{m}^2) (typical for neutrinos at this energy).
Neutrino Detection Efficiency: Assume 50% due to detector design and quantum efficiency of photodetectors.
Neutrino Production
Pions/Kaons Produced: [ N_{\text{pions/kaons}} = N_p \times 0.2 = 2 \times 10^{12} \text{ per second} ]
Neutrinos Produced: [ N_\nu = N_{\text{pions/kaons}} = 2 \times 10^{12} \text{ neutrinos per second} ]
Neutrino Flux at the Detector
Given the neutrinos spread out over a sphere: [ \text{Flux} = \frac{N_\nu}{4 \pi D^2} = \frac{2 \times 10^{12}}{4 \pi (100)^2} , \text{neutrinos/m}^2/\text{s} ] [ \text{Flux} \approx 1.6 \times 10^7 , \text{neutrinos/m}^2/\text{s} ]
Expected Interaction Rate in the Detector
Number of Target Nuclei ((N_t)) in the detector: [ N_t = 6 \times 10^{31} ]
Interactions per Second: [ R = \text{Flux} \times N_t \times \sigma \times \text{Efficiency} ] [ R = 1.6 \times 10^7 \times 6 \times 10^{31} \times 10^{-38} \times 0.5 ] [ R \approx 48 , \text{interactions/second} ]
Energy Deposited
Energy per Interaction: Assuming each neutrino interaction deposits roughly its full energy (150 MeV, or (150 \times 1.6 \times 10^{-13}) J): [ E_d = 150 \times 1.6 \times 10^{-13} , \text{J} = 2.4 \times 10^{-11} , \text{J} ]
Total Power: [ P = R \times E_d ] [ P = 48 \times 2.4 \times 10^{-11} , \text{J/s} ] [ P \approx 1.15 \times 10^{-9} , \text{W} ]
So, the power deposited in the detector from neutrino interactions would be about (1.15 \times 10^{-9}) watts.
Challenges and Improvements for Scaling Up
While the proof-of-concept might demonstrate the fundamental principles, scaling this up to transmit even a single watt of power, let alone megawatts, highlights the significant challenges:
Increased Beam Intensity: To increase the power output, the intensity of the proton beam and the efficiency of pion/kaon production must be dramatically increased. For high power levels, this would require a much higher energy and intensity accelerator, larger and more efficient targets, and more sophisticated focusing systems.
Larger Detector: The detector would need to be massively scaled
up in size. To detect enough neutrinos to convert to a practical amount of power, we're talking about scaling from a 10-ton detector to potentially tens of thousands of tons or more, similar to the scale of detectors used in major neutrino experiments like Super-Kamiokande in Japan.
Improved Detection and Conversion Efficiency: To realistically convert the interactions into usable power, the efficiency of both the detection and the subsequent energy conversion process needs to be near-perfect, which is far beyond current capabilities.
Steps to Scale Up the Experiment
To transition from the initial proof-of-concept to a more substantial demonstration and eventually to a practical application, several steps and advancements are necessary:
Enhanced Accelerator Performance:
Upgrade to Higher Energies: Move from a 500 MeV system to several GeV or even higher, as higher energy neutrinos can penetrate further and have a higher probability of interaction.
Increase Beam Current: Amplify the proton beam current to increase the number of neutrinos generated, aiming for a beam power in the range of hundreds of megawatts to gigawatts.
Optimized Target and Decay Tunnel:
Target Material and Design: Use advanced materials that can withstand the intense bombardment of protons and optimize the geometry for maximum pion and kaon production.
Magnetic Focusing: Refine the magnetic horns and other focusing devices to maximize the collimation and directionality of the produced neutrinos, minimizing spread and loss.
Massive Scale Detector:
Detector Volume: Scale the detector up to the kiloton or even megaton range, using water, liquid scintillator, or other materials that provide a large number of target nuclei.
Advanced Photodetectors: Deploy tens of thousands of high-efficiency photodetectors to capture as much of the light from interactions as possible.
High-Efficiency Energy Conversion:
Direct Conversion Technologies: Research and develop technologies that can convert the kinetic energy from particle reactions directly into electrical energy with minimal loss.
Thermodynamic Cycles: If using heat conversion, optimize the thermodynamic cycle (such as using supercritical CO2 turbines) to maximize the efficiency of converting heat into electricity.
Integration and Synchronization:
Data Acquisition and Processing: Handle the vast amounts of data from the detector with real-time processing to identify and quantify neutrino events.
Synchronization: Ensure precise timing between the neutrino production at the accelerator and the detection events to accurately attribute interactions to the beam.
Realistic Projections and Innovations Required
Considering the stark difference between the power levels in the initial experiment and the target power levels, let's outline the innovations and breakthroughs needed:
Neutrino Production and Beam Focus: To transmit appreciable power via neutrinos, the beam must be incredibly intense and well-focused. Innovations might include using plasma wakefield acceleration for more compact accelerators or novel superconducting materials for more efficient and powerful magnetic focusing.
Cross-Section Enhancement: While we can't change the fundamental cross-section of neutrino interactions, we can increase the effective cross-section by using quantum resonance effects or other advanced physics concepts currently in theoretical stages.
Breakthrough in Detection: Moving beyond conventional photodetection, using quantum coherent technologies or metamaterials could enhance the interaction rate detectable by the system.
Scalable and Safe Operation: As the system scales, ensuring safety and managing the high-energy particles and radiation produced will require advanced shielding and remote handling technologies.
Example of a Scaled Concept
To visualize what a scaled-up neutrino power transmission system might look like, consider the following:
Accelerator: A 10 GeV proton accelerator, with a beam power of 1 GW, producing a focused neutrino beam through a 1 km decay tunnel.
Neutrino Beam: A beam with a diameter of around 10 meters at production, focused down to a few meters at the detector site several kilometers away.
Detector: A 100 kiloton water Cherenkov or liquid scintillator detector, buried deep underground to minimize cosmic ray backgrounds, equipped with around 100,000 high-efficiency photodetectors.
Power Output: Assuming we could improve the overall system efficiency to even 0.1% (a huge leap from current capabilities), the output power could be: [ P_{\text{output}} = 1\text{ GW} \times 0.001 = 1\text{ MW} ]
This setup, while still futuristic, illustrates the scale and type of development needed to make neutrino power transmission a feasible alternative to current technologies.
Conclusion
While the concept of using neutrinos to transmit power is fascinating and could overcome many limitations of current power transmission infrastructure, the path from theory to practical application is long and filled with significant hurdels.
#Neutrino Energy Transmission#Particle Physics#Neutrino Beam#Neutrino Detector#High-Energy Physics#Particle Accelerators#Neutrino Interaction#Energy Conversion#Direct Energy Conversion#High-Voltage Direct Current (HVDC)#Experimental Physics#Quantum Materials#Nanotechnology#Photodetectors#Thermoelectric Generators#Superfluid Helium#Quantum Dots#Plasma Wakefield Acceleration#Magnetic Focusing Horns#Cherenkov Radiation#Scintillation Light#Silicon Photomultipliers (SiPMs)#Photomultiplier Tubes (PMTs)#Particle Beam Technology#Advanced Material Science#Cost-Effectiveness in Energy Transmission#Environmental Impact of Energy Transmission#Scalability of Energy Systems#Neutrino Physics#Super-Kamiokande
0 notes
Text
Liquid Scintillation Counter LSCL-A10 is a portable instrument controlled by SCM 8031, offers online data processing with sample capacity of 25 tubes and automatic change. Equipped with two position machine control; upper and lower position machine, can independently control the instrument sample change and measurement. With three working modes; count, DPM measurement, Quenched sample measurement, this device offers two curve fitting methods with curve fitting of the 3/2 degree equation; four parameter equation and abnormal point elimination function. This device can be used in the area to measure the intensity of low range β in the samples in 3H and 14C. Device is without computer setup, user have to use own computer setup.
0 notes
Text
A Wise Man Once Said
Precious lost its ring in the scrap yard with no metal detector the lavender pussywillows hide the trolls
Hong Kong wheel of fate UW spinned it first Knights of Templar slaughtered at a mass concert of bloody crimson tide
Tithe on a full moon for 2x the glee The crash of waves against the rocks, like bodies slapping against each other during sex blood shooting through veins Hot heat, sticky, in Iceland together I too, know of these lands
Tax season says the King! blue knots on a tent red food buckets hung like death #four crosses in a foreign land alone is no place to exist
An underwater welder lying on the blue tarp, is like a union of troops led by a zebra.
Flying flags at Disney welcome to the world of water failed regret, emptiness and betrayal tattered flags get left to rot sew it in with the others together and the quilt becomes strong and scintillating
Crush you with your own history headless horseman and halo hair dark horse donuts This is as good as it gets!
Red-lipped lipstick cracked porcelain face You can't hold a candle to this
King of the Hill My pool stick is clean now true Kings swim in the swimming pool together King of the Hill Jack of Spades went with the stolen crown and robots learn to volunteer.
Pledge to a sanitizer salute to a gong beat your chest it's loud and strong Love at first sight or sounds like a good idea Wisdom of the crowd or individual motivation?
A rabbi with the yachts Fortified lamps sees all UFOs, telekinesis and even explosive lingerie. One denarius for a days work Why they get more? Stand while another sits. Then switch roles and you'll see why.
What sees with three eyes? The melatonin-like parental bond, third eye awoken, Moksha.
Insane Luke has a scar red dots that kill. Baldie takes biosphere crown the bald animal is cutting loose again Is doraphilia still fun to you?
I attempt to transform but the tea is too strong my hands have small heart Lying down a tiny raindrop falls into my ear swirling into the cochlea My whole world has changed!
Eczema stealing make-up twice North Face go north Racks of weapons are not enough this time
My mask is old but gold bars had paved my fortunate path …a fortunate path(whispering)
Tik Tok vault one exit is enough The eagle has docked into spray-painted madness. Not to fret I hear a falcon cry Jump when the law is bent it will help you fly
Six shooter Six pack 3 sewers 3 fires Twin-spirit 1 spacesuit
Mountain top king of the hill climb Nepal Hajj pilgrimage princess climbs like a pirate piggyback down the wedding aisle
Opposites attract
One fell to its doom down the abyssal void towards the bottom and a ghost ship lost in the Bermuda Triangle with Pandoras Box Lazarus
Gunpowder in shoes Footprints in the sand Jesus did not tap
Short and tall fat and thin Lookalikes Soundalikes Smellalikes the hunt of touch and taste What double currencies create the ultimate Yin Yang effect? AI said to cure pride and competition, exchange abacus rubik-cubed calculators instead of cash.
Echoes and reverberation voices become lightning WATTS= AMPS X VOLTS
Float your payloads into the troposphere with skinny vertical structures of contained saltwater Heat a planet with a satellite asteroid belt
A call for help QR codes morse code gun flare smoke signal what are your coordinates? R-E-B-O-R-N
Some ancients say gunpowder only made flee then gun made to kill Oil spills from bronze age to silicon chips flood the market cut the mall castle cake in half Zangief on a segway You win.Perfect.
Lawrence Groves copyright©2024
74 notes
·
View notes
Text
today's affirmations: i am brave. i DO understand how scintillation detectors work. i understand my project methodology and can present it to people with ease.
28 notes
·
View notes
Text

Researchers develop perovskite X-ray detector for medical imaging
Shenzhen Institutes of Advanced Technology (SIAT) of the Chinese Academy of Sciences, in collaboration with researchers at Central China Normal University, have developed a high-performance perovskite X-ray complementary metal-oxide-semiconductor (CMOS) detector for medical imaging. The study was published in Nature Communications on Feb. 21. X-ray imaging is vital for the diagnosis and treatment of cardiovascular and cancer diseases. Direct-conversion X-ray detectors made of semiconductor materials exhibit superior spatial and temporal resolution at lower radiation doses compared to indirect-conversion detectors made of scintillator materials. However, the currently available semiconductor materials, such as Si, a-Se, and CdZnTe/CdTe, are not ideal for general X-ray imaging due to their low X-ray absorption efficiency or high costs.
Read more.
12 notes
·
View notes
Text

Missing link found in gamma emission phenomena from thunderclouds
Groundbreaking Discoveries in Gamma-Ray Emissions from Thunderstorms
In the recent edition of Nature, groundbreaking results about the gamma-ray emissions produced during thunderstorms are presented. Overall, these findings reveal that gamma-ray emission from thunderclouds is much more complex, diverse, and dynamic than previously thought. Understanding these phenomena is crucial to uncovering the secrets of lightning.
Flickering Gamma-Ray Flashes: A New Discovery
Entitled “Flickering Gamma-Ray Flashes, the Missing Link between Gamma Glows and TGFs,” the paper by Østgaard et al. [2024] reports unique observations of a new phenomenon called Flickering Gamma-Ray Flashes (FGFs).
During thunderclouds, two different hard radiation phenomena have so far been known to originate: Terrestrial Gamma-ray Flashes (TGFs) and gamma-ray glows. This third phenomenon, observed and named FGFs by Østgaard et al. [2024] resembles the other two, while at the same time revealing certain characteristics separating FGFs from the others. Most noteworthy may be that FGFs are pulses of gamma-rays not associated with any detectable optical or radio signals.
The Missing Link Found?
«We think that FGFs could be the missing link between TGFs and gamma-ray glows, whose absence has been puzzling the atmospheric electricity community for two decades», says lead author and Professor Nikolai Østgaard at the University of Bergen.
Dynamic Gamma-Ray Emissions in Tropical Thunderclouds
In another study presented in this edition of Nature, Marisaldi et al. [2024] focus on the phenomenon referenced above and known as gamma-ray glows. The paper, entitled «Highly dynamic gamma-ray emissions are common in tropical thunderclouds», shows that contrary to what has been believed until now, tropical thunderclouds over ocean and coastal regions commonly emit gamma rays for hours over areas up to a few thousand square kilometers.
A New Understanding of Gamma-Ray Glows
«The dynamics of gamma-glowing thunderclouds starkly contradicts the former quasi-stationary picture of glows, and resembles that of a huge gamma-glowing boiling pot both in pattern and behavior», says Professor Martino Marisaldi at the University of Bergen.
The ALOFT Campaign
The groundbreaking results presented by Østgaard et al. [2024] and Marisaldi et al. [2024] are based on observations from the ALOFT (Airborne Lightning Observatory for FEGS and TGFs) campaign.
ALOFT, a collaboration between NASA and the University of Bergen, involved flying the NASA ER-2 aircraft from the MacDill Air Force Base, Florida, over tropical thunderstorms around the Gulf of Mexico, Central America, and the Caribbean in the summer of 2023. The payload included lightning detectors, gamma-ray scintillators, and a mix of passive and/ or active microwave sensors. A total of 10 flights were conducted above thunderclouds around Mexico, El Salvador, Nicaragua, and Florida.
The ALOFT campaign was financed by the Research Council of Norway and led by Professor Nikolai Østgaard and Professor Martino Marisaldi from the University of Bergen, Timothy Lang (Marshall Space Flight Center, NASA), and Franzeska Becker (ER-2 Mission Management).
The instrumentation on Board ER-2 during the ALOFT campaign included:
Gamma-ray detectors UIB-BGO (PI: Nikolai Østgaard, UiB) and iStorm (PI: Eric Grove, NRL)
An optical instrument named FEGS (PI: Mason Quick, Marshall Space Flight Center, NASA)
Two electric field sensors known as EFCM (PI: Hugh Christian, University of Alabama, Huntsville) and LIP (PI: Christopher Schultz, Marshall Space Flight Center, NASA)
Four cloud characterization instruments: AMPR (PI: Timothy Lang, Marshall Flight Center, NASA), CRS and EXRAD (PI: Gerry Heymsfield, Goddard Space Flight Center, NASA), and CoSSIR (PI: Rachael Kroodsma, Goddard Space Flight Center, NASA)
The flight campaign was coordinated with ground-based radio observations managed by a large network of collaborators.
IMAGE: An illustration of NASAs research plane ER-2 flying over thunderstorms. Credit Artistic view of ALOFT observations by University of Bergen / Mount Visual,
6 notes
·
View notes
Text



Neutrino detectors, the underground cathedrals to science. The Japanese Super-Kamiokande and the Liquid Scintillator Neutrino Detector at Los Alamos
3 notes
·
View notes
Text
A lot of houses legit have a problem with their concrete basements being thick with radioactive radon daughter products. In some areas a high-than-normal rate of lung cancer can be directly blamed on common building materials, frequent temperature inversions, or the types of naturally occurring rocks. You can read more about this from the EPA. Of course if you want to check your basement for radon a RADIAC meant for detecting general area radiation levels is not the instrument you want to use, an air sampler and an alpha particle detector would be better. You can detect alpha with a Geiger-Muller tube, but I've always used a scintillator myself. So if you have readings on that thing then, yeah, it's probably more likely to be fallout than radon.
Or maybe someone was making dirty bombs. Or maybe Marie Curie used to live there. Or maybe it's a whole lot of really old watches.
workplace comedy sketch where a nuclear power engineer and a paranormal investigator get their equipment mixed up and go to their respective jobs unaware of the difference


#PSA about basement radon#If your basement is concrete and you experience frequent temperature inversions GET IT TESTED#I mean it radon daughter products can give you lung cancer#Radon is literally the 2nd most common cause for lung cancer behind smoking
41K notes
·
View notes
Text
0 notes
Text
Advanced Radiation Detection Drones: Enhancing Safety and Precision in Hazardous Environments
In recent years, the integration of drone technology into various fields has revolutionized how we approach complex and hazardous tasks. One of the most significant advancements is the development of radiation detection drones. These sophisticated machines are transforming the way we handle radiation monitoring and management, offering unprecedented safety and precision in hazardous environments. This article explores how advanced radiation detection drones work, their benefits, and their applications in enhancing safety and efficiency.
Understanding Radiation Detection Drones
Radiation detection drones are unmanned aerial vehicles (UAVs) equipped with specialized sensors to detect and measure radiation levels from a safe distance. These drones can be outfitted with a variety of radiation sensors, including gamma detectors, scintillation counters, and dosimeters, allowing them to identify different types of radiation such as alpha, beta, and gamma rays. The primary goal of these drones is to monitor and assess radiation levels in environments that are dangerous or difficult for human operators to access.
Key Benefits of Radiation Detection Drones
1. Enhanced Safety
One of the most compelling advantages of the best radiation detection drones is their ability to enhance safety for both workers and the public. In environments with high radiation levels, such as nuclear power plants, radioactive waste sites, or areas affected by nuclear accidents, human exposure to radiation can be extremely dangerous. Drones can enter these hazardous areas and collect critical data without putting human lives at risk. By remotely assessing radiation levels, these drones help prevent unnecessary exposure and ensure that safety protocols are strictly followed.
2. Improved Precision and Accuracy
Advanced radiation detection drones are equipped with highly sensitive and precise sensors that provide accurate radiation measurements. The ability to collect data from various altitudes and angles enhances the precision of radiation mapping and assessment. Drones can create detailed radiological maps that show radiation hotspots and contamination levels with high resolution, which is crucial for making informed decisions about decontamination, evacuation, and safety measures.
3. Rapid Response and Real-Time Monitoring
In emergency situations, such as nuclear accidents or radiation leaks, rapid response is critical. Radiation detection drones can be deployed quickly to assess the situation and provide real-time data on radiation levels. This immediate feedback enables emergency responders to make swift decisions and implement effective containment and mitigation strategies. The real-time monitoring capabilities of these drones also facilitate ongoing surveillance of radiation levels, ensuring that changes are detected promptly and that appropriate actions are taken.
4. Cost-Effectiveness
Using drones for radiation detection can be more cost-effective compared to traditional methods. Deploying human teams to manually monitor radiation levels in hazardous environments involves significant costs related to protective gear, training, and health risks. Drones reduce these costs by minimizing the need for direct human involvement in dangerous areas. Additionally, drones can cover large areas more efficiently than ground-based methods, potentially reducing the time and resources required for radiation monitoring.
Applications of Radiation Detection Drones
1. Nuclear Power Plants
Nuclear power plants are critical infrastructure that require constant monitoring to ensure safety. Radiation detection drones are used to inspect reactor buildings, cooling systems, and spent fuel storage pools. They can detect radiation leaks, assess the condition of containment structures, and monitor radiation levels in areas that are difficult to access. By providing detailed data, drones help operators maintain safety standards and address potential issues before they become critical.
2. Radioactive Waste Management
The management of radioactive waste involves handling materials that remain hazardous for extended periods. Radiation detection drones play a vital role in monitoring waste storage facilities and assessing potential contamination. They can inspect waste storage containers, track the movement of waste materials, and ensure that safety protocols are being followed. This continuous monitoring helps prevent leaks and contamination, safeguarding both the environment and public health.
3. Environmental Monitoring
Radiation detection drones are also used for environmental monitoring to assess the impact of radiation on natural ecosystems. After nuclear accidents or during routine environmental assessments, drones can survey affected areas, measure radiation levels in soil, water, and vegetation, and track changes over time. This information is crucial for understanding the extent of contamination and implementing remediation efforts.
4. Disaster Response
In the event of a nuclear or radiological disaster, drones provide critical support for disaster response teams. They can quickly assess the affected area, identify radiation hotspots, and guide response efforts. Drones equipped with thermal imaging and other sensors can also help locate victims and assess damage in contaminated environments, improving the efficiency of rescue operations.
Future Trends and Developments
As technology continues to advance, radiation detection drones are expected to become even more sophisticated. Future developments may include:
Increased Sensor Sensitivity: Enhancements in sensor technology will improve the detection capabilities of drones, allowing them to measure even lower levels of radiation with greater accuracy.
Enhanced Autonomy: Advances in artificial intelligence and machine learning will enable drones to perform more complex tasks autonomously, such as analyzing radiation data and making real-time decisions.
Extended Range and Endurance: Improvements in battery technology and drone design will extend the operational range and endurance of radiation detection drones, allowing them to cover larger areas and perform longer missions.
Conclusion
Advanced radiation detection drones represent a significant leap forward in the field of radiation monitoring and management. By offering enhanced safety, precision, and efficiency, these drones are transforming how we handle hazardous environments and respond to emergencies. Their ability to provide real-time data, reduce costs, and improve safety makes them an invaluable tool for a wide range of applications, from nuclear power plants to environmental monitoring. As technology continues to evolve, radiation detection drones will play an increasingly
1 note
·
View note
Video
youtube
MEG II's Pixelated Timing Counter
The MEG II (Mu2e Experiment at Fermilab II) is an experiment designed to search for the rare muon-to-electron conversion process, which is a powerful probe for new physics beyond the Standard Model. One of the key components of the MEG II experiment is the Pixelated Timing Counter (PTC), which plays a crucial role in improving the experiment's sensitivity and precision. Pixelated Timing Counter (PTC) Purpose: The Pixelated Timing Counter is used to provide precise timing information for the detection of charged particles. Its primary role is to time-stamp particle hits with high accuracy, which is essential for distinguishing between signal and background events in the experiment.
Design and Features:
Pixelated Structure: The PTC consists of a large array of small, pixel-like detectors that are arranged in a grid. Each pixel can detect the arrival time of a particle with high resolution. Timing Resolution: The PTC is designed to achieve very fine time resolution, often in the range of picoseconds. This allows for the precise measurement of particle arrival times and helps in distinguishing closely spaced events. Performance: The pixelated design enhances the ability to track and time particles accurately over a large area. This is critical for experiments like MEG II, where precise timing and spatial resolution are required to measure rare decays and distinguish them from background noise. Technology: The PTC often uses advanced detector technologies such as scintillators coupled with photodetectors or other fast timing devices. These technologies are selected based on their ability to provide high timing resolution and reliability. Importance in MEG II: The PTC significantly improves the experiment’s sensitivity by reducing the background noise and increasing the precision in timing measurements. This, in turn, helps in the accurate measurement of the rare muon-to-electron conversion process and improves the overall data quality of the experiment. By providing precise timing information, the Pixelated Timing Counter enhances the ability of the MEG II experiment to probe new physics scenarios and search for processes that are otherwise extremely challenging to detect.
More Info: physicistparticle.com
contact us : [email protected]
#youtube#megii#pixelatedtimingcounter#timingresolution#particlephysics#muontoelectronconversion#timingdetector#highenergyphysics#scintillator#precisetiming#fermilab
0 notes
Text
0 notes
Text
Understanding X-Ray Detectors for Digital Radiography: A Comprehensive Guide
Digital radiography (DR) has revolutionized the field of medical imaging, offering enhanced image quality, faster processing times, and reduced radiation exposure compared to traditional film-based systems. At the heart of this technological advancement lies the X-ray detector, a critical component that captures the X-rays passing through the body and converts them into digital images. This blog delves into the world of X-ray detectors for digital radiography, exploring their types, working principles, and the benefits they bring to modern healthcare.
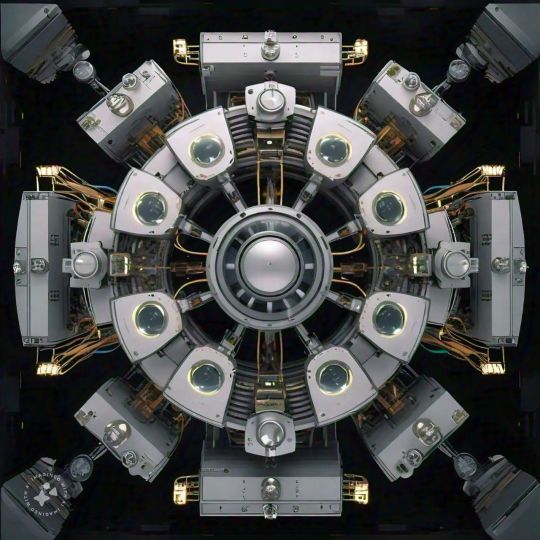
What Are X-Ray Detectors?
X-ray detectors are devices that capture and convert the X-ray energy emitted from an X-ray source into visible images. These images allow healthcare professionals to diagnose and monitor various medical conditions, ranging from broken bones to complex diseases. In digital radiography, the role of the X-ray detector is crucial as it directly influences the image quality, resolution, and overall efficiency of the imaging process.
Download PDF Brochure
Types of X-Ray Detectors in Digital Radiography
There are several types of X-ray detectors used in digital radiography, each with its unique advantages and applications:
Flat-Panel Detectors (FPDs):
Indirect Conversion Detectors: These detectors use a scintillator to convert X-rays into visible light, which is then detected by photodiodes and converted into electrical signals. Indirect conversion FPDs are widely used in general radiography due to their excellent image quality and relatively low radiation dose.
Direct Conversion Detectors: These detectors directly convert X-ray photons into electrical signals without the intermediate step of light conversion. Made from materials like amorphous selenium, direct conversion FPDs offer higher spatial resolution, making them ideal for applications requiring detailed imaging, such as mammography.
Charged-Coupled Devices (CCDs): CCDs are semiconductor devices that convert X-rays into electrical signals. Though less commonly used than FPDs, CCDs are still prevalent in specific applications like dental imaging. They offer good image quality but are often limited by their smaller size and lower sensitivity compared to FPDs.
Complementary Metal-Oxide-Semiconductor (CMOS) Detectors: CMOS detectors are similar to CCDs but use different technology for capturing and processing X-ray images. CMOS detectors are known for their low power consumption, high speed, and durability. They are increasingly used in various medical imaging applications, including portable X-ray systems.
Photostimulable Phosphor Plates (PSP): Also known as computed radiography (CR) detectors, PSP plates store the X-ray image in a phosphor layer, which is later read out by a laser scanner. Although CR systems are less expensive than FPDs, they are gradually being replaced by more advanced digital radiography technologies due to their slower processing times.
Key Advantages of Digital Radiography with X-Ray Detectors
The transition from traditional film-based radiography to digital radiography has brought numerous benefits, largely due to advancements in X-ray detector technology:
Improved Image Quality: X-ray detectors in digital radiography provide superior image quality with better contrast resolution, enabling more accurate diagnoses.
Reduced Radiation Exposure: Digital X-ray detectors require less radiation to produce high-quality images, which enhances patient safety.
Request Sample Pages
Faster Results: The digital nature of modern X-ray detectors allows for immediate image processing and review, significantly speeding up the diagnostic process.
Enhanced Workflow Efficiency: Digital radiography systems are integrated with Picture Archiving and Communication Systems (PACS), enabling seamless storage, retrieval, and sharing of images within healthcare networks.
Environmentally Friendly: Unlike film-based systems, digital radiography eliminates the need for chemical processing and film storage, reducing environmental impact.
Conclusion
X-ray detectors are the cornerstone of digital radiography, transforming how medical images are captured, processed, and analyzed. With the ongoing advancements in detector technology, digital radiography continues to evolve, offering ever-improving image quality, patient safety, and diagnostic accuracy. As healthcare providers strive to deliver better care, understanding the different types of X-ray detectors and their benefits is essential for making informed decisions in medical imaging.
Content Source:
0 notes
Text
X-ray Scintillator Market Size, Analyzing Forecasted Outlook and Growth for 2024-2030
Global Info Research announces the release of the report “Global X-ray Scintillator Market 2024 by Manufacturers, Regions, Type and Application, Forecast to 2030” . The report is a detailed and comprehensive analysis presented by region and country, type and application. As the market is constantly changing, the report explores the competition, supply and demand trends, as well as key factors that contribute to its changing demands across many markets. Company profiles and product examples of selected competitors, along with market share estimates of some of the selected leaders for the year 2024, are provided. In addition, the report provides key insights about market drivers, restraints, opportunities, new product launches or approvals, COVID-19 and Russia-Ukraine War Influence.
The scintillator is the core component of the X-ray detector, it is the key to the sensitivity and resolution of the X-ray detector, and it is also a class that can convert high-energy photons or particles (X-rays, gamma rays, alpha particles, etc.) Luminescent materials with energy visible photons.
X-ray Scintillator is the material work by converting X-ray energy into a near visible or visible light.
According to our (Global Info Research) latest study, the global X-ray Scintillator market size was valued at US$ 216 million in 2023 and is forecast to a readjusted size of USD 292 million by 2030 with a CAGR of 4.2% during review period.
Global core manufacturers of X-ray scintillator include Proterial (Hitachi Metals), Luxium Solutions (Saint-Gobain Crystals), Toshiba Materials, Dynasil, Meishan Boya Advanced Materials, and Shanghai SICCAS. The top three manufacturers account for Approximately 36% global share.
China and North America are the world's largest markets, accounting for nearly 56% of the market share. In terms of product types, inorganic scintillator occupies a dominant position, accounting for 81%. At the same time, in terms of applications, industry inspection is the largest application field, accounting for 49%.
This report is a detailed and comprehensive analysis for global X-ray Scintillator market. Both quantitative and qualitative analyses are presented by manufacturers, by region & country, by Type and by Application. As the market is constantly changing, this report explores the competition, supply and demand trends, as well as key factors that contribute to its changing demands across many markets. Company profiles and product examples of selected competitors, along with market share estimates of some of the selected leaders for the year 2024, are provided. Key Features:
Global X-ray Scintillator market size and forecasts, in consumption value), sales quantity, and average selling prices, 2019-2029
Global X-ray Scintillator market size and forecasts by region and country, in consumption value, sales quantity, and average selling prices, 2019-2029
Global X-ray Scintillator market size and forecasts, by Type and by Application, in consumption value, sales quantity, and average selling prices, 2019-2029
Global X-ray Scintillator market shares of main players, shipments in revenue, sales quantity, and ASP, 2019-2024
The Primary Objectives in This Report Are:
To determine the size of the total market opportunity of global and key countries
To assess the growth potential for X-ray Scintillator
To forecast future growth in each product and end-use market
To assess competitive factors affecting the marketplace
This report profiles key players in the global X-ray Scintillator market based on the following parameters - company overview, production, value, price, gross margin, product portfolio, geographical presence, and key developments.
The report involves analyzing the market at a macro level: Market Sizing and Segmentation: Report collect data on the overall market size, including the sales quantity (K Units), revenue generated, and market share of different by Type: Organic Scintillator Inorganic Scintillator Industry Analysis: Report analyse the broader industry trends, such as government policies and regulations, technological advancements, consumer preferences, and market dynamics. This analysis helps in understanding the key drivers and challenges influencing the X-ray Scintillator market. Regional Analysis: The report involves examining the X-ray Scintillator market at a regional or national level. Report analyses regional factors such as government incentives, infrastructure development, economic conditions, and consumer behaviour to identify variations and opportunities within different markets. Market Projections: Report covers the gathered data and analysis to make future projections and forecasts for the X-ray Scintillator market. This may include estimating market growth rates, predicting market demand, and identifying emerging trends. The report also involves a more granular approach to X-ray Scintillator: Company Analysis: Report covers individual X-ray Scintillator manufacturers, suppliers, and other relevant industry players. This analysis includes studying their financial performance, market positioning, product portfolios, partnerships, and strategies. Consumer Analysis: Report covers data on consumer behaviour, preferences, and attitudes towards X-ray Scintillator This may involve surveys, interviews, and analysis of consumer reviews and feedback from different by Application: Medical Industrial Inspection Military & Defense Others
Technology Analysis: Report covers specific technologies relevant to X-ray Scintillator. It assesses the current state, advancements, and potential future developments in X-ray Scintillator areas. Competitive Landscape: By analyzing individual companies, suppliers, and consumers, the report present insights into the competitive landscape of the X-ray Scintillator market. This analysis helps understand market share, competitive advantages, and potential areas for differentiation among industry players. Market Validation: The report involves validating findings and projections through primary research, such as surveys, interviews, and focus groups.
The Main Contents of the Report, includes a total of 15 chapters:
Chapter 1, to describe Optical Tweezers (Mechanobiology Equipment) product scope, market overview, market estimation caveats and base year.
Chapter 2, to profile the top manufacturers of Optical Tweezers (Mechanobiology Equipment), with price, sales, revenue and global market share of Optical Tweezers (Mechanobiology Equipment) from 2019 to 2024.
Chapter 3, the Optical Tweezers (Mechanobiology Equipment) competitive situation, sales quantity, revenue and global market share of top manufacturers are analyzed emphatically by landscape contrast.
Chapter 4, the Optical Tweezers (Mechanobiology Equipment) breakdown data are shown at the regional level, to show the sales quantity, consumption value and growth by regions, from 2019 to 2030.
Chapter 5 and 6, to segment the sales by Type and application, with sales market share and growth rate by type, application, from 2019 to 2030.
Chapter 7, 8, 9, 10 and 11, to break the sales data at the country level, with sales quantity, consumption value and market share for key countries in the world, from 2017 to 2023.and Optical Tweezers (Mechanobiology Equipment) market forecast, by regions, type and application, with sales and revenue, from 2025 to 2030.
Chapter 12, market dynamics, drivers, restraints, trends and Porters Five Forces analysis.
Chapter 13, the key raw materials and key suppliers, and industry chain of Optical Tweezers (Mechanobiology Equipment).
Chapter 14 and 15, to describe Optical Tweezers (Mechanobiology Equipment) sales channel, distributors, customers, research findings and conclusion.
The analyst presents a detailed picture of the market by the way of study, synthesis, and summation of data from multiple sources by an analysis of key parameters. Our report on the X-ray Scintillator market covers the following areas:
X-ray Scintillator market sizing
X-ray Scintillator market forecast
X-ray Scintillator market industry analysis
Analyze the needs of the global X-ray Scintillatorbusiness market
Answer the market level of global X-ray Scintillator
Statistics the annual growth of the global X-ray Scintillatorproduction market
The main producers of the global X-ray Scintillatorproduction market
Describe the growth factor that promotes market demand
Global Info Research is a company that digs deep into global industry information to support enterprises with market strategies and in-depth market development analysis reports. We provides market information consulting services in the global region to support enterprise strategic planning and official information reporting, and focuses on customized research, management consulting, IPO consulting, industry chain research, database and top industry services. At the same time, Global Info Research is also a report publisher, a customer and an interest-based suppliers, and is trusted by more than 30,000 companies around the world. We will always carry out all aspects of our business with excellent expertise and experience.
0 notes
Text
0 notes