We don't know where we're going, but we'll get there fast.
Don't wanna be here? Send us removal request.
Text
Albert Einstein’s general theory of relativity
100 years ago today Albert Einstein presented his general theory of relativity to the Prussian Academy of Science. It was the result of eight years of brain-bending work.
The equations set out in his theory describe how gravitational fields of matter affect space and time. They predict that the fields can change not just space, but also time, causing it to become curved. His theory has had a wide-ranging impact – from being one of the cornerstones of modern cosmology, to an integral element in the invention of modern GPS, of which we currently feel the benefits of on our smartphone and other devices.
To celebrate the 100 year anniversary since the publication of Einstein’s general theory of relativity, we’ve pulled together a list of articles below:
’Relativity’ from Magic Universe: A Grand Tour of Modern Science
‘On Einstein’s Theory of Gravitation and its Astronomical Consequences. First Paper’ from Monthly Notices of the Royal Astronomical Society
‘General Relativity and Einstein’s Equations’ from General Relativity and the Einstein Equations
‘Discussion on the Theory of Relativity’ from Monthly Notices of the Royal Astronomical Society
‘Cosmology’ from Einstein’s Physics: Atoms, Quanta, and Relativity - Derived, Explained, and Appraised
‘Expanding Galactic Systems and a New Application of Einstein’s General Theory of Relativity’ from Monthly Notices of the Royal Astronomical Society
‘Einstein, Albert’ from The Oxford Companion to Cosmology
Image: Spacetime lattice analogy, by Mysid. CC-BY-SA-3.0 via Wikimedia Commons.
309 notes
·
View notes
Photo
After Black Holes Collide, a Puzzling Flash
A surprising burst of light appeared in the sky at the same time as a collision between two black holes. Is the flash just a cosmic coincidence, or will it force astrophysicists to rethink what black holes can do?
On Sept. 14, 2015, at almost the exact same time that a pair of sprawling gravitational-wave detectors heard the last gasp of a collision between two black holes, another, more perplexing observation took place. Over 500 kilometers above the surface of the Earth, the orbiting Fermi Gamma-Ray Space Telescope logged a passing burst of gamma rays, a high-energy form of light. The signal was so slight that the NASA scientists who run the satellite didn’t notice it at first.
“[The gravitational-wave detector] LIGO saw a bright event, clear in their data, and we found a little blip in our data that’s really only credible because it happened so close in time to the gravitational wave,” said Valerie Connaughton, a member of the Fermi team.
On Feb. 11, the Fermi researchers posted a paper to the scientific preprint site arxiv.org describing the gamma-ray burst and speculating that it likely originated from the same black-hole merger that produced the gravitational waves observed by LIGO (the Laser Interferometer Gravitational-Wave Observatory). The correlation, which is far from certain, would upend entrenched assumptions in physics. Astrophysicists have long believed that black holes exist in a vacuum, as they tend to swallow up all nearby matter. This absence of matter means it should be impossible for two merging black holes to generate a flash of light.
“If you don’t have charged particles, you don’t have magnetic fields, and you can’t get electromagnetic radiation,” said Adam Burrows, an astrophysicist at Princeton University. “It’s too clean a system.”
But the gamma-ray burst detected by the Fermi satellite suggests that perhaps the neighborhood around a pair of black holes isn’t so empty after all. In the days since the Fermi team released their paper, a number of astrophysicists have hastened to propose theoretical explanations for how matter might persist around black holes in high enough concentrations to generate a gamma-ray burst. These theories involve flights of astrophysical imagination, pulled together in the wake of a historic event, to explain an observation of light that by all accounts should not have been there.
A Cosmic Coincidence?
Gamma rays fall at the very end of the electromagnetic spectrum. Of all the varieties of light, they have the shortest wavelengths, the highest frequency and the most energy — millions of times more energy than ultraviolet light, for example.
It takes extreme conditions to create that much energy, and only two known astrophysical events might do so. One is the collapse of a massive star into a black hole. As the stellar core falls in on itself, it shucks off its surrounding envelope of matter and forms violent jets of energy that propel that matter out into space at nearly the speed of light. These are the so-called “long gamma-ray bursts,” which account for about 80 percent of all gamma-ray bursts and typically last around 20 seconds.
The second mechanism for producing a gamma-ray burst is the merger of two very compact objects, such as a pair of neutron stars or a neutron star and a black hole. In the case of a star and a black hole, matter from the star forms a ring of material called an accretion disk around the black hole. As the material from the accretion disk falls into the black hole, jets of energy form along the axis of the merger. The result is a “short gamma-ray burst,” which typically lasts less than two seconds.
Gamma-ray bursts are the great pyrotechnic events of the universe, explosions on a scale that we can hardly imagine. They also provide astrophysicists with a way of seeing hidden cosmic events.
“Short gamma-ray bursts allow us to view dark objects,” Connaughton said. “When [these objects] merge, they produce a violent jet of energetic particles, and we see the violence in a phenomenon that would otherwise look very dark.”
On Sept. 14, Fermi detected a short, transient event that registered as a blip. It was so dim that the team did not even notice it at first. Later, when they learned that LIGO had detected a gravitational wave, they went back through their data to see if Fermi had seen anything interesting at the same time. Using an algorithm developed by Lindy Blackburn, an astronomer at the Harvard-Smithsonian Center for Astrophysics in Cambridge, Mass., and a member of the LIGO team, the Fermi researchers searched for faint blips in their noisy data. That’s when they saw it, a burst of gamma rays that arrived 0.4 seconds after the gravitational wave and lasted one second. It had characteristics of a typical short gamma-ray burst that, at its origin, contained 10,000 trillion times the amount of energy the sun produces over that same length of time.
Whether the gamma-ray burst was real, rather than a detection error, and whether, if real, it’s connected to the LIGO event has become a topic of intense debate in the weeks since the Fermi team published their paper.
The team has roughly established that the gamma-ray burst came from a 2,000-square-degree region of the sky. Combined with the 600-degree LIGO localization, the arrival direction is reduced to a 200-square-degree patch of sky, supporting the conclusion that the gamma-ray burst and the gravitational waves originated in the same place. The timing of the two events suggests this as well. Fermi detects blips of this magnitude about once every 10,000 seconds (or about every 2 hours and 47 minutes), making it unlikely, although not impossible, that the near-simultaneous observation of the gamma-ray burst and the gravitational waves was a coincidence.
“It’s a low-chance possibility, but it’s not impossible that this happened by chance,” Connaughton said. “That’s why we’re circumspect about claiming this is a counterpart to the LIGO event. It’s a ‘three-sigma’ result, not something we’d take to the bank under normal circumstances.” In fact, at the same time that Fermi noted the burst, another gamma-ray detector, the European Space Agency’s Integral satellite, observed nothing. “From our perspective, it is quite unlikely the event Fermi has detected is related to the gravitational-wave event,” said Carlo Ferrigno, a member of the Integral team.
More fundamentally, the Fermi team is being cautious about linking the two events because the merger of two black holes is simply not supposed to generate light. “Everything is in its favor, except for physics, which is a problem,” Connaughton said.
The physics does pose a problem — or at least a riddle.
“To produce a gamma-ray burst you need some conventional matter like an accretion disk around the merging object,” said John Ellis, a particle physicist at King’s College London. “I think it’s pretty clear if you’re talking about the merger of neutron stars you’d have that matter. It’s not so obvious around black holes.”
The accuracy of Fermi’s observation will be resolved over time. LIGO will presumably detect more gravitational waves. As it does so, the Fermi team will look for corresponding gamma-ray bursts. If they find them, they’ll know they’re onto something.
Building Bright Black Holes
In the meantime, astrophysicists have been trying to explain how there could be enough material around a pair of black holes to produce a gamma-ray burst. Bing Zhang, an astrophysicist at the University of Nevada, Las Vegas, has speculated that if one or both of the merging black holes contained a charge, that charge might be sufficient to create a magnetic field that could generate a gamma-ray burst. But according to the general consensus, astrophysical black holes should have no measurable charge.
Another proposal comes from Rosalba Perna, an astrophysicist at Stony Brook University. In a paper posted to arxiv.org on Feb. 16, she and two colleagues speculate that two massive stars locked together in a binary star system might both die, forming two black holes. As the second massive star in the system dies, debris from its envelope might fall back toward the core and settle into an accretion disk. Then, as a merger begins, the companion black hole would enter the other through this disk, powering a gamma-ray burst.
Avi Loeb, the chairman of the astronomy department at Harvard University, has offered a third possibility. In a paper posted to arxiv.org on Feb. 15 and subsequently accepted for publication in The Astrophysical Journal Letters, Loeb describes how a pair of black holes might originate simultaneously inside a star 100 times as large as the sun. As he envisions it, this massive star was originally created when two smaller stars combined. The conditions of that merger cause the massive star to spin very rapidly. When it eventually begins to collapse, the centrifugal force from the spin causes its core to break into two clumps in a dumbbell configuration, and each clump forms a black hole — with the two black holes gravitationally intertwined inside the remnants of the massive star.
“It’s sort of like a pair of twins in the belly of a pregnant mother, and as they come together, they make one black hole,” Loeb says.
The black holes in Loeb’s scenario eventually merge, and because the merger takes place inside a massive star, there would be plenty of material around to fuel a gamma-ray burst — in fact, Loeb imagines that as much as a whole solar mass would fall into the newly created black hole per second at the time of the merger.
Loeb’s paper is only the beginning of an effort to explain an observation that, if it holds up, would demand a new way of thinking from astrophysicists. A rapidly spinning, supermassive star of the kind at the center of his proposal has never been seen. Additionally, in scenarios where a star has a rapidly rotating inner core, the core doesn’t usually split into two dumbbells — it creates a flattened disc with spiral arms. Over the next year, Loeb and others will run computer simulations to determine whether it’s possible to generate the conditions described in his paper. Some of Loeb’s colleagues are skeptical that his scenario will end up working.
“Personally, I think this is a bit of a stretch,” said Burrows. “There are a few tooth fairies that have been concatenated here to explain what may be a spurious detection.”
Others think that Loeb’s paper points the field of astrophysics in the right direction, regardless of whether it ends up being correct.
“As always in science when there are important new discoveries, in this case LIGO, there’s a time of early speculation where people throw out ideas,” said Volker Bromm, an astrophysicist at the University of Texas, Austin. “I think Avi’s paper is excellent because it focuses people’s attention on what needs to be done. It’s definitely plausible.”
In time, the authenticity of the Fermi detection will become clear. If it does prove accurate, theories will eventually develop that explain how two black holes create a gamma-ray burst. They may resemble the ideas that have been proposed by Zhang, Perna and Loeb, or they may end up looking completely different. What’s clear is that post-LIGO, there is a lot of new science to be done. The rush to untangle the implications of the post-gravitational-wave world is already under way.
Image Credit: davidope
183 notes
·
View notes
Text
What are Gravitational Waves?
Today, the National Science Foundation (NSF) announced the detection of gravitational waves by the Laser Interferometer Gravitational-Wave Observatory (LIGO), a pair of ground-based observatories. But…what are gravitational waves? Let us explain:

Gravitational waves are disturbances in space-time, the very fabric of the universe, that travel at the speed of light. The waves are emitted by any mass that is changing speed or direction. The simplest example is a binary system, where a pair of stars or compact objects (like black holes) orbit their common center of mass.
We can think of gravitational effects as curvatures in space-time. Earth’s gravity is constant and produces a static curve in space-time. A gravitational wave is a curvature that moves through space-time much like a water wave moves across the surface of a lake. It is generated only when masses are speeding up, slowing down or changing direction.
Did you know Earth also gives off gravitational waves? Earth orbits the sun, which means its direction is always changing, so it does generate gravitational waves, although extremely weak and faint.
What do we learn from these waves?
Observing gravitational waves would be a huge step forward in our understanding of the evolution of the universe, and how large-scale structures, like galaxies and galaxy clusters, are formed.
Gravitational waves can travel across the universe without being impeded by intervening dust and gas. These waves could also provide information about massive objects, such as black holes, that do not themselves emit light and would be undetectable with traditional telescopes.

Just as we need both ground-based and space-based optical telescopes, we need both kinds of gravitational wave observatories to study different wavelengths. Each type compliments the other.
Ground-based: For optical telescopes, Earth’s atmosphere prevents some wavelengths from reaching the ground and distorts the light that does.
Space-based: Telescopes in space have a clear, steady view. That said, telescopes on the ground can be much larger than anything ever launched into space, so they can capture more light from faint objects.
How does this relate to Einstein’s theory of relativity?
The direct detection of gravitational waves is the last major prediction of Einstein’s theory to be proven. Direct detection of these waves will allow scientists to test specific predictions of the theory under conditions that have not been observed to date, such as in very strong gravitational fields.
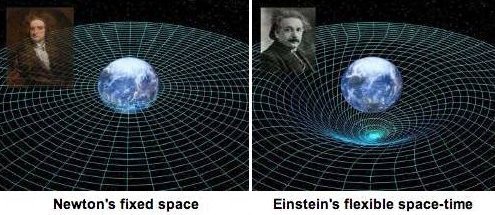
In everyday language, “theory” means something different than it does to scientists. For scientists, the word refers to a system of ideas that explains observations and experimental results through independent general principles. Isaac Newton’s theory of gravity has limitations we can measure by, say, long-term observations of the motion of the planet Mercury. Einstein’s relativity theory explains these and other measurements. We recognize that Newton’s theory is incomplete when we make sufficiently sensitive measurements. This is likely also true for relativity, and gravitational waves may help us understand where it becomes incomplete.
Make sure to follow us on Tumblr for your regular dose of space: http://nasa.tumblr.com
7K notes
·
View notes
Photo
Scientists from the Laser Interferometer Gravitational-Wave Observatory (LIGO) will make a major research announcement on Thursday, at 10:30 a.m. Eastern Time, at a press conference hosted by the National Science Foundation. Unfortunately, the press conference is for media only, so there will be no live streaming events. Nevertheless, we’re skeptically excited about tomorrow. If the rumors are true, and gravitational waves have been discovered, it would mean that the most elusive prediction of Einstein’s theory of relativity is right, gravitational radiation exists, and that these waves can be detected. Finding gravitational waves would be a monumental scientific discovery, it would kick-start a new era for gravitational-wave astronomy!
Gravitational waves explained by physicists Umberto Cannella and Daniel Whiteson. (video)
Image Credit: S. Larson
897 notes
·
View notes
Text
The EPR Paradox
Einstein-Podolsky-Rosen Paradox (Podolsky and Rosen were associates of Einstein.)

Einstein not being impressed with what quantum mechanics did there.
The EPR paradox challenges how quantum theory can be related to physical properties, and is a challenge to quantum entanglement.
Remember the Heisenberg Uncertainty Principle (HUP)? The point being that within quantum mechanics, physical properties of the classical nature (position, momentum, spin, etc.) are not able to be defined at the same time with any sort of precision. So, let’s say we’re talking about the spin of a particle that resides in three dimensions along the axes x, y, and z. We’re checking out one of these particles and are able to determine that the particle is spinning down on the z axis. That’s cool, but we cannot possibly know what’s going down in the other two axes (HUP!!!).
Hold the phone, friends. What if we had two photons that were stuck together (and had the property of zero angular momentum), then broke those photons into two separate states? We can now define those systems by the same quantum state due to their initial identicalness. These particles are entangled particles, and will be defined as having opposite spins (up/down) (remember!?) so as to cancel each other out, and produce the zero angular momentum that was present in the initial system. In order to determine the spin of the first photon, we had to disturb the system, and therefore, we caused the spin to form (HUP!). The point of this is that we can know what’s going on with the second photon without even checking it out, because we know what’s going on with the first photon. We didn’t even measure it!
Do you know what that means? It means that the second photon HAD THAT SPIN ALL ALONG. And do you know what that means? It means that the photon “KNEW” WHAT THE FIRST PHOTON WOULD BECOME BEFORE WE DISTURBED THE SYSTEM!!!!
Pardon my enthusiasm. Einstein did not like this freaky shit going down about particles “predicting” things (also how it sort of didn’t sit right with his very own special theory of relativity, surely a matter of personal pride). So he and his posse postulated that there were ~*HIDDEN VARIABLES*~ that should reconcile the problems. It goes down like this. Either
1) Quantum theory supports instantaneous information transfer (or prediction of certain outcomes without observation), regardless of distance (NON-LOCALITY!!!).
or
2) Quantum theory is not complete, and there are hidden variables (of the physical nature, ~ow ow) that account for the information transfer. The variables would not violate physical properties of the classical sense (LOCALITY!!!).
Sooooooo, now that we know the spin of the second photon due to the first photon, we can now measure what’s going down on another axis. And so that would mean that we know for a FACT two separate physical properties, and that violates the HUP!!! Hence, the paradox.
Of course, this … misunderstanding could be due to our limited knowledge of quantum mechanics. But it’s still MIGHTY interesting (see: MIND-BLOWING) to think about.
28 notes
·
View notes
Video
youtube
3.5 years of philosophizing later, I think I’ve linked gravity (as a non-fundamental force), why the speed of light is fixed, and Classical Newtonian physics. Is it delusions of grandeur, or something worth thinking about? I know it’s long (TWSS) at 1 hr 15 mins, but I just am too tired and do not have enough time in the day to boil it down any further, at present. I do have to work a full time job to pay the bills! ;) TL;DR - Dimensionality of space should be defined by the Higgs field, which vibrates. Therefore space itself should be vibrating. Such a vibration should therefore lead to convection of quantum particles, which is what we perceive as gravity.
#submission#everyone watch this and comment#I'm writing a dissertation and won't have time to think about anything but cardiac muscle for the next six months#alas#i bet this is fucking great tho
11 notes
·
View notes
Photo
Like any self-respecting American, I am Wikipedia-ing at school and came across this fun fact. Walter Bishop was a consultant for Project MKUltra! If Wiki says it, it must be true, right?
12 notes
·
View notes
Note
So recently in my physics class, we learned about sound waves and how they work. Well other than being a bit of a nerd, I love playing video games, specifically space simulators. In a few of these games, you can hear ships fly by you as well as their engines and guns. So my question is, could sound pass into your ship's atmosphere allowing you to hear things in space? Or would sound be completely stopped by the vacuum of space? Love your blog! And thanks. :P
The background here is that sound waves are mechanical waves which require a medium to propagate. Unlike electromagnetic waves which can travel in a vacuum.
The thing to understand is that mechanical waves don’t just “need a medium to propagate” the wave is the oscillation of matter and so is part of the medium. Ripples in a lake are energy transfer between water molecules and could not exist without the water.
Mechanical waves are created by some energy disturbance that gets transferred from one particle to the next. The particles don’t have to move position, only the energy moves from one place to another.
So back to your question: if your spaceship had some sort of force-field of matter surrounding it in the vacuum of space, you could conceivably hear anything that came inside that force-field. So long as that matter was good enough at transmitting sound energy that the energy would make it to you over the distances involved. But only then.
You would also likely be able to hear anything that hit your ship as your ship is mad of matter and could transmit the sound of impact like a gong. But the ship that shot you would hear nothing.
If you were fighting inside a gas cloud you might hear something, but it would be very distorted and short-range. Gas is not a great transmitter of sound energy. Shout outside and see how far your voice carries, now think how close your space ships would have to be to hear each other scream.
79 notes
·
View notes
Video
youtube
Traveling Waves
Paul Andersen explains how traveling waves move through space and time. The reflection and interference of traveling waves can create standing waves which appear motionless. Examples of traveling waves in one and two dimensions are included.
By: Bozeman Science. Support at: http://www.bozemanscience.com/donate/
36 notes
·
View notes
Quote
Every action is a movement of energy. The wave on the ocean is not The movement of water. It is the movement of Energy through the water, The movement of The Animator through the inanimate. To see this clearly is to Understand the workings of the universe. When there is understanding of The workings of the universe, There can be no Resistance or opposition. When there is no Resistance or opposition. All that remains is peace.
The Lost Writings of Wu Hsin (via subcognition)
156 notes
·
View notes
Photo

What is a “False Vacuum” and are we living in one?
In any physical system you’ll find that there’s an energy “ground state” that the system tries to approach. For example, if you pour water into a bathtub there are a lot of ways that the water could arrange itself, but it will rapidly try to assume the ground state: being as low and still as possible.
Gravitational potential (the energy that something on a high shelf has more of, and that’s released when things fall) is the easiest example of an energetic system to picture. Being on the ground is the lowest gravitational potential a thing can have (without digging); thus the name.
But in the tub example, the water in the tub doesn’t “know” about the drain or the area around the tub. Given the chance the water would flow out of the tub and into a new, lower, ground state. In fancy-math-speak, you’d say that the water in a tub is in a “local energy minimum”. Within the tub, water definitely assumes the only ground state it can find. However, if it jumped out of the tub, or somebody pulled the plug, then it would try to find a new, lower ground state and would find that it gained a bunch of new energy in the process (what with the flowing and splashing and whatnot).
The idea of energy levels, and ground states, and all that, applies to pretty much everything. That includes electromagnetic fields and even particle fields. A “particle field” is the quantum mechanical way of describing particles (all smeared out, instead of being all in one point), and each field has a pretty reasonable set of energy levels. Every new particle elevates the energy level by one step, and the ground level is exactly what you’d expect: zero particles.
The vacuum is the most absolute ground state: no waves, no particles, nothing at all to elevate the energy above zero*. However, all the dynamics of the universe are governed bydifferences in energy level. For example, when you fill a tub it doesn’t matter if the tub is at the top of a mountain, or at the bottom of a mine, the water will behave the same way. So, the idea behind a false vacuum is that what we consider the ground state of the universe isn’t really the ground state, and it may be possible to drop into an even lower-energy state (drain the tub, so to speak). What we think is the ground state, the vacuum, may not be the true ground state. So it’s called a “false vacuum”.
The “danger” of living in a false vacuum is that, under the proper circumstances the false vacuum can drop into the true vacuum. The cause is usually described as a sufficient burst of energy to get the appropriate fields “over the hump” (picture above). If the difference in energy between the false vacuum and true vacuum is large enough, then the surrounding space can likewise be tipped into the lower state. In theory, a “false vacuum collapse” would expand at light speed (or about light speed) from the originating event, and destroy the heck out of everything in the affected, and ever-expanding, region.
read more
200 notes
·
View notes
Photo
It's not often I get to reblog my other self.


There are stranger and more stunning things than gold in the deep places of the world.
For more than five decades, scientists have descended deep beneath the Black Hills of South Dakota to hunt for subatomic treasures. The pioneers of neutrino-hunting, led by our own Nobel Prize-winning Ray Davis, used the cavernous Homestake mine to filter out unwanted particles and track ghostly interactions with massive detectors. That bold work of the 1960s continues today in state-of-the-art underground laboratories.
Symmetry magazine just dove into the rich history and exciting future of particle physics in the Homestake mine. Check it out!
295 notes
·
View notes
Photo
Quantum Tunneling
Quantum tunneling refers to the quantum mechanical phenomenon where a particle tunnels through a barrier that it classically could not surmount. This plays an essential role in several physical phenomena, such as the nuclear fusion that occurs in main sequence stars like the Sun. It has important applications to modern devices such as the tunnel diode, quantum computing, and the scanning tunneling microscope. The effect was predicted in the early 20th century and its acceptance as a general physical phenomenon came mid-century.
Tunneling is often explained using the Heisenberg uncertainty principle and the wave–particle duality of matter. Pure quantum mechanical concepts are central to the phenomenon, so quantum tunneling is one of the novel implications of quantum mechanics.
source
4K notes
·
View notes
Video
youtube
Seeing the Smallest Thing in the Universe
Beyond molecules and atoms, how far down can we see below the wavelength of visible light? What is the smallest possible scale in the universe?
By: Physics Girl. Support at: http://j.mp/SupportPhysicsGirl
46 notes
·
View notes
Photo

Are the Quantum World and The Real World the Same Thing?
By Andrew Friedman
While quantum mechanics is arguably our most successful theory of nature, it is perhaps best known for its strangeness. Quantum theory—and its key mathematical tool, the wave function—excels at predicting probabilities for the outcomes of experiments. Yet, after nearly a century of debate, physicists and philosophers of science can agree only that there is no real consensus on what quantum theory actually says about the world. This has led to a cottage industry of interpretations of quantum theory, which now number in the hundreds if not the thousands.
At the center of this quagmire is the “wave function.” Using the wave function, better known by its mathematical nickname, ψ (“psi”), physicists can calculate the probability that a quantum measurement will have a particular outcome. The success of this procedure has allowed us to control the subatomic world with unprecedented precision: You can thank (or curse) quantum theory for your iPads, smartphones, and laptops. Yet, unlike classical physics, quantum mechanics can’t deliver a single, definite answer to a simple question about the outcome of a measurements. Instead, it returns a probability distribution representing many different possible outcomes. It’s only after you make a measurement that you observe a stable, predictable, classical outcome. At this point, the wave function is said to have “collapsed.”
Continue Reading
272 notes
·
View notes